Hypothesis and Theory: Fungal Spores in Stemflow and Potential Bark Sources
- 1National Public Health Center, Budapest, Hungary
- 2Applied Coastal Research Laboratory, Georgia Southern University, Savannah, GA, United States
- 3Geology and Geography, Georgia Southern University, Savannah, GA, United States
- 4Biosciences, Mangalore University, Mangalore, India
- 5Centre for Environmental Studies, Yenepoya University, Mangalore, India
The study of stemflow fungi began over 50 years ago. Past work has been performed in different climatic regions of the world, with different sampling methods, by mycologists focusing on different taxonomical groups. Therefore, we aim to synthesize this work to delineate major conclusions and emerging hypothesis. Here, we present: (1) a systematic compilation of observations on stemflow conidial concentration, flux, and species composition; (2) an evaluation of the methods underlying these observations; (3) a testable theory to understand spatiotemporal dynamics in stemflow (including honeydews) conidial assemblages, with a focus on their relationship to bark structure and microhabitats; and (4) a discussion of major hypotheses based on past observations and new data. This represents a knowledge gap in our understanding of fungal dispersal mechanisms in forests, in a spatially-concentrated hydrologic flux that interacts with habitats throughout the forest microbiome. The literature synthesis and new data represent observations for 228 fungal species’ conidia in stemflow collected from 58 tree species, 6 palm species, and 1 bamboo species. Hypothetical relationships were identified regarding stemflow production and conidial concentration, flux, and species composition. These relationships appear to be driven by bark physico-chemical properties, tree canopy setting, the diversity of in-canopy microenvironments (e.g., tree holes, bark fissures, and epiphytes), and several possible conidia exchange processes (teleomorph aerosols, epi-faunal exchanges, fungal colonization of canopy microhabitats, and droplet impacts, etc.). The review reveals a more complex function of stemflow fungi, having a role in self-cleaning tree surfaces (which play air quality-related ecoservices themselves), and, on the other hand, these fungi may have a role in the protection of the host plant.
Introduction
During precipitation or condensation, tree canopies capture and drain water down the undersides of branches. These branchflows redirect water from outlying canopy areas to concentrated drip points (Van Stan et al., 2020a), to treeholes [creating isolated aquatic habitats called “dendrotelmata” (Magyar et al., 2017b)], or multiple branchflows may converge at the stem to create “stemflow” (Sadeghi et al., 2020). Branchflows and stemflow can play important roles within the canopy and in receiving ecosystems. Within tree canopies, branchflows can enable exchanges between the tree’s external and internal microbial communities (Aung et al., 2018), or spread pathogens through resident animal communities (e.g., D’Amico and Elkinton, 1995). Branchflows, and their suspended and dissolved constituents, that contribute to dendrotelmata can affect mosquito control and plant health (Carpenter, 1982; Van Stan et al., 2020b). Before stemflow reaches the ground, it may serve as a drinking water source for canopy-dwelling animals, e.g., koalas (Mella et al., 2020). Stemflow that reaches the surface can supply substantial, localized water (Magliano et al., 2019), nutrient (Ponette-González et al., 2020), pollutant (Klučiarová et al., 2008), and organismal fluxes to the litter and soils (Sridhar, 2009; Bittar et al., 2018; Ptatscheck et al., 2018). When stemflow is able to preferentially infiltrate into the subsurface along root channels (Friesen, 2020), it can interact with the rhizosphere (Johnson and Jost, 2011; Rosier et al., 2015), and influence bedrock-soil interactions (Backnäs et al., 2012).
Because stemflow can influence ecohydrological processes throughout the critical zone, the materials carried by stemflow merit research attention regardless of its typically small proportion (<2%) of gross rainfall in natural forests (Van Stan and Gordon, 2018). As stemflow drains through the canopy, it primarily scours the bark surface, dissolving or suspending, and transporting materials on (and within) that surface. Bark surfaces are structurally complex, enabling it to scavenge aerosolized particles (Suzuki, 2006), and some types and sizes of particulates are more effectively scavenged by bark than by leaves (Xu et al., 2019). Bark surfaces are colonized by a wide range of “corticolous” epiphytes, including plants (Mendieta-Leiva et al., 2020), metazoans (Proctor et al., 2002), and microbes (Akinsoji, 1991; Magyar, 2008; Lambais et al., 2014). Waste from phloem-feeding canopy residents, called “honeydews,” can be sticky and nutritive (Miller et al., 1994; Shaaban et al., 2020), affecting both aerosol scavenging and the bark residential community (Dhami et al., 2013). Thus, stemflow may encounter a diverse array of organic and inorganic materials and, indeed, a diversity of solutes and particulates have been observed in stemflow in ecologically relevant amounts (Ponette-González et al., 2020). Dissolved organic carbon concentrations in stemflow, for example, represent some of the highest observations to-date in natural waters (Stubbins et al., 2020). The flux of nematodes and tardigrades within stemflow can be 105 individuals year–1 tree–1, and even larger for rotifers, ∼106 individuals year–1 tree–1 (Ptatscheck et al., 2018). Fungal spores, or conidia, have been observed in stemflow at even higher concentrations, 101–103 conidia 10-mL–1, resulting in an annualized flux of ∼109 conidia ha–1 year–1 (Gönczöl and Révay, 2004; Sridhar and Karamchand, 2009; Van Stan et al., 2021).
Fungal conidia are non-motile and, therefore, rely on environmental processes, like wind or water flows, for their mobilization. Conidia in stemflow have been observed to be produced, liberated, and dispersed by fungi in synchrony with storms (MacKinnon, 1982). Stemflow-dispersed conidia are branched or filiform; these “staurospores” and “scolecospores” are well-structured for transport in the thin, rivulet-like stemflow pathways (Bandoni and Koske, 1974; Chauvet et al., 2016).
Although more descriptive studies of conidia have been published than any other particulate in stemflow to-date (Ponette-González et al., 2020), there has been no review of these observations in pursuit of a theory to explain, and test hypotheses regarding the spatial and temporal dynamics in the concentration, flux or species composition of stemflow conidial assemblages. Here, we present: (1) a systematic compilation of observations on stemflow conidial concentration, flux and species composition; (2) an evaluation of the methods underlying these observations; (3) a testable theory to understand spatiotemporal dynamics in stemflow (including honeydews) conidial assemblages, with a focus on their relationship to bark structure and microhabitats; and (4) a discussion of major hypotheses based on past observations and new data. This represents a knowledge gap in our understanding of fungal dispersal mechanisms in forests, in a spatially-concentrated hydrologic flux that interacts with habitats throughout the forest microbiome (Van Stan et al., 2021). This effort also addresses recent calls for improved ecological understanding of tree-fungi interactions (Uroz et al., 2016) and fungal spore dispersal within the forest microbiome (Baldrian, 2017).
Methods
Literature Synthesis
This metaanalysis used data compiled from a synthesis of published studies that reported species of free fungal conidia observed on bark surfaces, in honeydews, and in stemflow (Supplementary Table 1). Several databases were searched (Web of Science, BIOSIS, Current Contents Connect, and The Scientific Electronic Library Online) without a date restriction (i.e., 1864-present) for “conidi∗” AND “stemflow” OR “bark” OR “honeydew.” Search results were pared down to only those studies that reported observations, of at least presence or absence, for conidial assemblages (i.e., studies of a single fungal species were excluded) from intact trees (not logs, stumps, and debris, etc.) that were healthy (i.e., not with studies on phytopathogenic fungi, like Fusarium or Septoria spp.). We then searched for other woody/rigid plants (woody vines, palms and bamboo) with the same search settings. Digital data on conidial assemblages that were available directly from study authors were compiled into a single database. Digitization of conidia species data in the remaining studies was done using Tabula 1.2.11. To ensure that the Tabula software did not incorrectly digitize the datasets, a random 25% of observations in each table were checked manually. The only Tabula errors encountered were related to mismatched column-row information and these formatting errors were corrected when found. Some studies reported the abundance or frequency of observation for conidia species; in these cases, the data were transformed into presence or absence to enable study inter-comparison. As stemflow, bark and honeydew conidia assemblages are less-researched than for other habitats, several unknown conidia have been found across studies. Microscopic imagery of unknown conidia was compared across studies to determine (1) if the conidia has been identified and (2) if unknown conidia across studies were morphologically similar. Note that bark, stemflow and honeydew data can be from different studies and, thus, may not have been sampled synchronously. However, this is the data available per the authors’ knowledge and the literature search described above. The host tree species were recorded for all cases where the information was provided. When the bark texture of sampling tree was described, it was also recorded. Tree species without bark textural descriptions were classed based on taxonomic descriptions or photographic reference materials. The bark textural classes are listed alongside a photographic representation in Figure 1. These classes include scaled or plated bark, fissured bark, ridged bark flaked or exfoliating bark, smooth bark, and external plant tissues on tall, non-tree vegetation, analogous to bark: palm “pseudobark,” and bamboo culm (Figure 1).
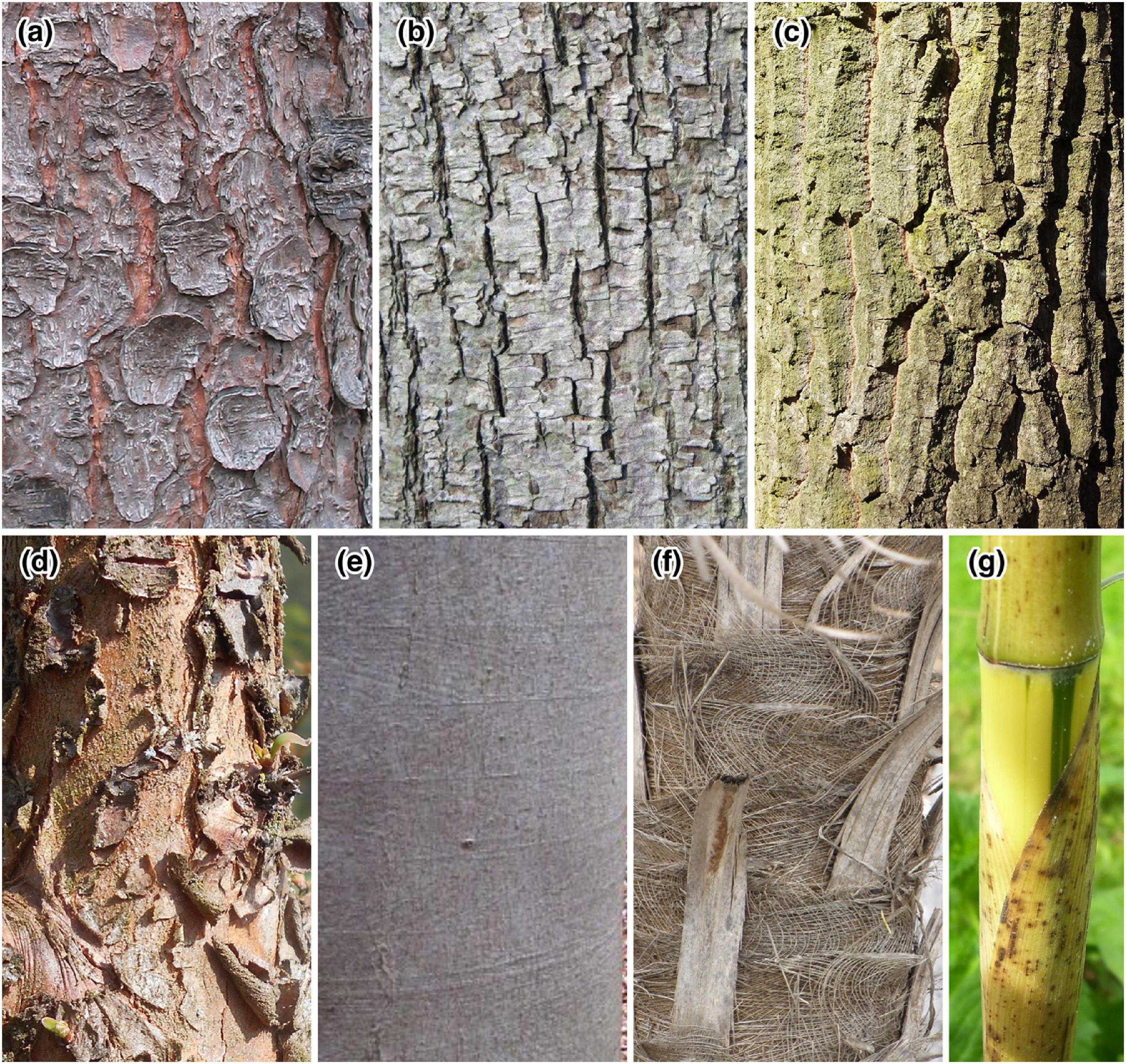
Figure 1. Bark types included in studies to-date examining fungal conidia assemblages in stemflow, bark or honeydew: (a) scaled or plated bark, e.g., Picea abies (Kwiecień, 2005); (b) fissured bark, e.g., Tilia cordata (Havelaar, 2020); (c) ridged bark, e.g., Quercus cerris (Lefnaer, 2016); (d) flaked or exfoliating bark, e.g., Metasequoia glyptostroboides (Salicyna, 2017); (e) smooth bark, e.g., Fagus sylvatica (Elsner, 2012); (f) palm “pseudobark,” e.g., Coccothrinax barbadensis (Starr and Starr, 2009); and (g) bamboo culm, e.g., Phyllostachys vivax (Hanfmampf, 2008).
Collection and Identification of New Conidia Assemblages
To supplement the database of published conidia assemblages in stemflow, bark and honeydews, additional data from ongoing field studies by the lead author were included in the database. Sampling and conidia identification methods (as described below) were similar to those from previously published studies. These new data include conidial assemblages identified from bark, stemflow and honeydew samples (Supplementary Table 2), which were added to the database. Augmentation of published datasets with these new data was done to provide the most comprehensive synthesis available to date for building theory and major hypotheses. These new data permitted better replication of conidial assemblages from existing bark types, honeydew and stemflow. No individual analysis, theoretical discussion, or related hypothesis relied solely on new data.
Stemflow samples were collected directly from the stem by diverting a stemflow rivulet (2–10 mL) into centrifuge tubes. One milliliter of FAA (50% ethanol, 5% glacial acetic acid, 10% formaldehyde) was added to each sample (Ingold, 1975). Water samples were settled; one drop of the sediment was mounted on a microscope slide and allowed to dry. Lactophenol cotton blue was added to the dried sediment to prepare samples for further studies.
Bark samples were collected from living trees in Hungary, then the surface and fissures were analyzed using pressure-sensitive acrylic strips (MACbond B 1200, MACtac Europe S.A., Brussels). The strip consists of a thin (20 μm) polypropylene film coated on both sides with a rubber-based adhesive, which offers very high adhesion property on wood (Similar methods are widely used in building inspection for molds as well as in clinical mycology). Bark fissures were opened by force using a scalpel put deep into the cracks. A 1.5 cm2 piece of strip was placed on the opened surface of the fissure, then it was pressed against it and peeled. Lactophenol with cotton blue was added to the sampling side of the strip, which was then covered with a cover slip to prepare semi-permanent slide preparations for further studies. Three-to-four preparations were made from each bark sample.
Honeydew is an extract from piercing and plant-sucking insects, which suck phloem sap, which is rich in nutrients, especially amino acids. To satisfy their protein needs, these insects need large amounts of sap, which contains only 1–2% of proteins, though it is high in water content and sugars. When honeydew production is high on forest trees, honeydew drops fall to the ground or flow down on stems. Animals, mostly birds, ants, wasps and bees (i.e., Apis mellifera L.) often feed on honeydew. Honeybees collect and transport it to hives and process it into honeydew honey (often sold and labeled as forest honey). Due to the collecting activities of honeybees the spores trapped in the honeydew will therefore accumulate in honeydew honey. Thus, 10 g were sampled from 500 g of previously homogenized honey, dissolved in 20 ml of distilled water at 40°C, centrifuged for 10 min at 560 g and allowed to settle. The sediment was recovered in 10 ml of distilled water and again centrifuged. The sediment was then collected with a Pasteur pipette and dried onto microscope slides at 40°C. It was then mounted in glycerine-gelatine and covered (Louveaux et al., 1978).
The tapes and slides prepared from all samples were viewed directly under a microscope to identify the types of spores present on the sampled surface. Identification of fungal spores was carried out both from experience and by means of scientific literature and monographs (e.g., Hughes, 1958; Ingold, 1971, 1975; Kendrick, 1990; Ellis and Ellis, 1997; Marvanová, 1997; Gulis et al., 2007). Digital photomicrographs were taken with an Olympus BX-51 microscope at ×800 magnification.
An Evaluation of Stemflow Conidia Sampling Methods
A description and discussion of the methods used to collect stemflow samples for the quantification and identification of conidial assemblages is necessary to identify any non-trivial limitations surrounding the observations in this synthesis and evaluation. For stemflow, the first samples to be examined for conidia were taken from the foam that can accumulate at the base of some trees (Gönczöl, 1976: Figure 2a); however, stemflow sampling has most often been done during storms through the direct transfer of stemflow from the bark surface (e.g., Figure 2b) to a collector containing preservative (Gönczöl and Révay, 2004, 2006; Sridhar and Karamchand, 2009; Sudheep and Sridhar, 2010; Ghate and Sridhar, 2015c; Magyar et al., 2018). Rarely have stemflow samples been collected after storms from collection bins (Bandoni, 1981; MacKinnon, 1982). Although sampling from a collection bin after storms has been the norm for stemflow hydrology and solute fieldwork (Levia and Germer, 2015), this post-storm sampling method can introduce species that have colonized the plastic tubing and collection bins between storms. Another limitation of this method is that spores germinate rapidly in water samples and morphological identification and counting can become impossible. Bulk post-storm stemflow sampling allows researchers to collect one sample that integrates the intra-storm variability and all stemflow rivulets around the tree stem (note that many stemflow pathways can travel from the canopy to the surface: Figures 2b,c). Conversely, direct sampling of stemflow rivulets into clean or sterilized collectors with preservative during a storm gains a representative snapshot of the conidial assemblage with the least possibility of contamination. This snapshot sampling, however, must be repeated (1) throughout a storm (e.g., Gönczöl and Révay, 2004) to account for the conidial assemblages’ intra-storm variability or (2) for all stemflow rivulets around a tree to account for the spatial variability (Figure 2c).
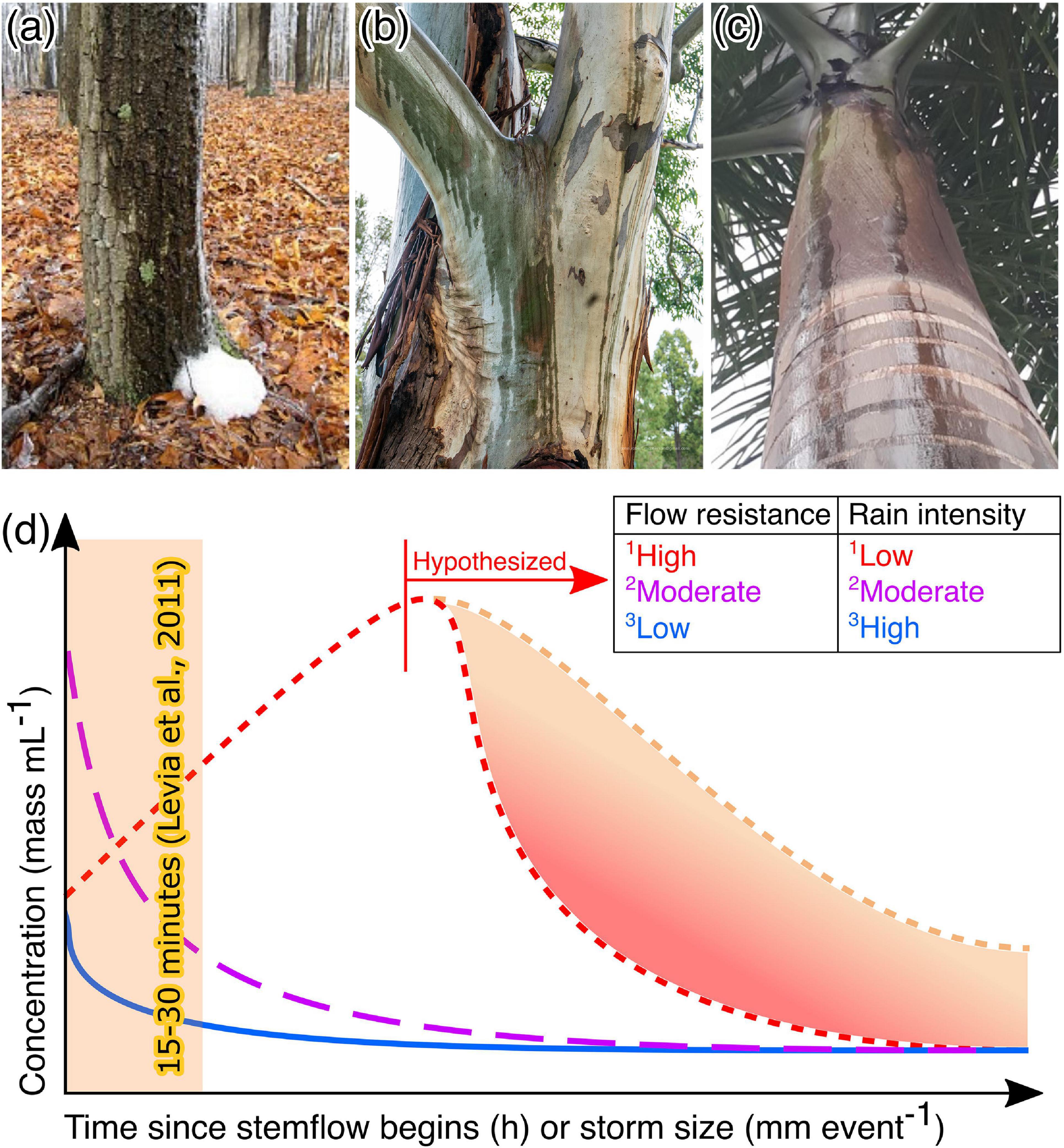
Figure 2. Photographs showing types of stemflow that has been sampled, including (a) the foam that can sometimes accumulate at the base of a stemflow rivulet (West, 2010); (b) major stemflow rivulets (McPherson, 2018); and (c) multiple discrete stemflow rivulets on a single trunk of a palm, Roystonea regia [photograph reproduced from Salemi (2019) with permission]. (d) Conceptual summary of different temporal dynamics previously observed in stemflow solute concentrations from different combinations of tree species and rain intensities. 1Quercus virginiana hosting a substantial bromeliad-fern-lichen epiphyte assemblage in subtropical maritime forest, Georgia, United States (Van Stan et al., 2017). 2Liriodendron tulipifera and 3Fagus grandifolia, both in a temperate, maritime forest, Delaware, United States (Levia et al., 2011). Note that no new data was used to generate this conceptual summary.
To date, the experimental designs for sampling stemflow have not included samples across multiple storm conditions, seasons, or years. Based on theory and past related literature, it may be hypothesized that there is significant intra-storm and seasonal variability in stemflow conidia concentration. Observations of stemflow solutes within storms and among storms of various magnitudes suggest that the timing of any snapshot sampling of stemflow rivulets during the storm can be important (Levia et al., 2011; Van Stan et al., 2020a). Interactions between rainfall intensity and a tree canopy’s resistance to stemflow generation (due to high water storage capacity or rough bark, etc.) can result in differing dynamics of materials out-washed by stemflow (Figure 2d). For example, typically stemflow rivulets are sampled 15–30 min after stemflow is established (Sridhar and Karamchand, 2009; Sudheep and Sridhar, 2010; Ghate and Sridhar, 2015c)—depending on the storm characteristics and the structure of the tree being sampled, this single sampling event could capture (a) the initial “wash off” pulse of conidia for a moderately intense storm over a canopy which moderately resists flow (Figure 2d, magenta), (b) a point along the gradual wetting and washing off period for a low intensity storm over a canopy with high flow resistance (Figure 2d, red), or (c) miss the wash off pulse entirely and capture the more dilute, post-wash off period for a high intensity storm over a canopy which permits flow (Figure 2d, blue). Some trees with canopies that have very high flow resistance may not have generated stemflow yet, despite all other trees having done so. Thus, these trees would not be able to be sampled by the snapshot approach, for example: see the lack of stemflow data on Chamaecyparis or Taxus tree species in Gönczöl and Révay (2004, 2006).
Less observational evidence is available to hypothesize on the compositional variability that one may expect among different stemflow rivulets. Still, theoretically, to ensure that spatial variability of conidial transport and assemblage composition around the stem is accounted for, multiple stemflow rivulets (Figures 2b,c) should be sampled for conidia. Indeed, it is likely that different stemflow rivulets integrate different areas of the canopy—from different aspects, exposures (windward v. leeward), or microenvironments (dendrotelma overflows v. branchflows). The number of discrete stemflow rivulets may change with rainfall intensity—see photographs in Levia et al. (2011)—but dye tests suggest that these rivulets over the bark surface are highly preferential (Imamura et al., 2017) and exhibit non-uniform flow patterns over time (Tischer et al., 2020). The thin sheet-like flow structure of stemflow also makes direct sampling for conidia via syringes challenging, especially during low rainfall intensities. Gönczöl and Révay (2004) commented on this sampling challenge, stating that “during heavy rain when the stemflow was copious the syringe could easily be filled … However, during low intensity rain a lesser quantity of water, sometimes 1 or 2 ml, could only be collected.” Some studies have addressed the spatial issue by having collected stemflow samples across a trunk area using sterile plastic sheets (Sridhar and Karamchand, 2009; Sudheep and Sridhar, 2010; Ghate and Sridhar, 2015c).
Stemflow Conidia Concentrations and Flux Estimates
To the authors’ knowledge, few studies report the concentration and flux of stemflow conidia (Gönczöl and Révay, 2006; Sridhar and Karamchand, 2009; Ghate and Sridhar, 2015c) and no studies to date have comprehensively assessed the size of the conidial reservoir on-and-in bark and honeydews, or the temporal variability of these bark conidial sources to stemflow. We also lack an understanding of variability in stemflow conidial concentration, flux and composition across temporal scales as no studies report trends across storms, seasons or years (see previous section). The snapshot data available, however, include several species with disparate bark and canopy structures situated within temperate sites (Germany, Hungary, Romania, and Sweden: Gönczöl and Révay, 2006) and a tropical monsoon site (Mangalore, India: Sridhar and Karamchand, 2009; Ghate and Sridhar, 2015c). No new data was available/added regarding conidia concentrations and fluxes.
The tree species studied across temperate European sites included Fagus sylvatica, Prunus avium, Carpinus betulus, Alnus glutinosa, Quercus cerris, Taxus baccata, Picea abies, and Pinus sylvestris (Gönczöl and Révay, 2006). For these temperate tree species, total conidia concentrations in stemflow ranged from approximately 2,000–16,000 conidia L–1. The maximum stemflow conidia concentration reported for temperate trees was observed from an evergreen needleleaved tree, T. baccata, while the minimum was observed from a deciduous broadleaved tree, Q. cerris (Gönczöl and Révay, 2006). One of the possible drivers of these differences in stemflow conidia concentration may be the amount of stemflow that study trees were able to generate: T. baccata generated very low stemflow volumes compared to Q. cerris (Gönczöl and Révay, 2006). This comparison of the minimum and maximum observations, however, provides a limited insight into differences among major tree types. For example, although an evergreen needleleaved species generated the largest stemflow conidia concentration across temperate sites, the conidia concentration from P. sylvetris, another needleleaved evergreen species, was nearly as low (3,100 conidia L–1) as observed from Q. cerris (Gönczöl and Révay, 2006). Perhaps the larger conidia concentration from T. baccata, specifically, may be due to the bark chemical composition and affinity to store water—this latter may be partially explained by a more complex, exfoliating bark structure compared to the other trees studied. Resins may also play an important role in shaping the stemflow conidia concentration, where, for example, Mycoceros colonization of pine and spruce species (whose bark contains resin droplets that keep the bark dry) was low (Révay and Gönczöl, 2011b; Magyar et al., 2017a). Moreover, a range of growth inhibitory compounds have been reported from the wood of Pinus species (Erdtmann, 1952; Scheffer and Cowling, 1966; Gunasekera and Webster, 1983) that may suppress fungal colonization and sporulation.
An important caveat regarding the Gönczöl and Révay (2006) stemflow conidia concentrations is that, for four of the sampled trees, some spore species were not counted due to being too “numerous.” For A. glutinosa and F. sylvatica, only 1 of the trees had unquantified results and could be ignored due to data being provided from other individual trees of the same species. This was not the case for Q. cerris. As a result, conidia concentrations can be considered underestimates for Q. cerris. Despite the underestimates for some trees in the Gönczöl and Révay (2006) study, their stemflow conidia concentration results compare well with those from the tropical monsoon studies.
A greater number of species have been investigated at the tropical monsoon site with regards to stemflow conidia concentrations, including trees: Acacia auriculiformis, Alstonia scholaris, Artocarpus integrifolia, Carallia brachiata, Careya arborea, Eucalyptus tereticornis, Ficus benghalensis, Ficus religiosa, Mangifera indica, Odina wodier, Pongamia glabra, Syzygium cumini, Tectona grandis, and Terminalia paniculata (Sridhar and Karamchand, 2009); and palms: Areca catechu, Borassus flabellifer, Caryota urens, Cocos nucifera, Livistona rotundifolia, and Roystonea regia (Ghate and Sridhar, 2015c). For the tree species, stemflow conidia concentrations ranged from 4,800–52,600 conidia L–1 (Sridhar and Karamchand, 2009). Interestingly, just as observed in Gönczöl and Révay (2006), the highest conidia concentration in stemflow from the tropical monsoon site was observed from a tree species with a complex, exfoliating bark structure: T. grandis. The lowest stemflow conidia concentration from any tree sampled by Sridhar and Karamchand (2009) was by the evergreen broadleaved tree, M. indica. For the palm species, the range of stemflow conidia concentrations was an order of magnitude lower: 230–5,790 conidia L–1 (Ghate and Sridhar, 2015c). Therefore, the lowest observation for stemflow conidia concentrations was reported for a palm species, Roystonea regia. To examine whether trends emerge in stemflow conidia concentrations across plant types or with variability in stemflow production, the reported stemflow conidia concentrations were grouped per plant type (Figure 3A) and, where possible, plotted against published observations of the species’ stemflow fraction (Figure 3B).
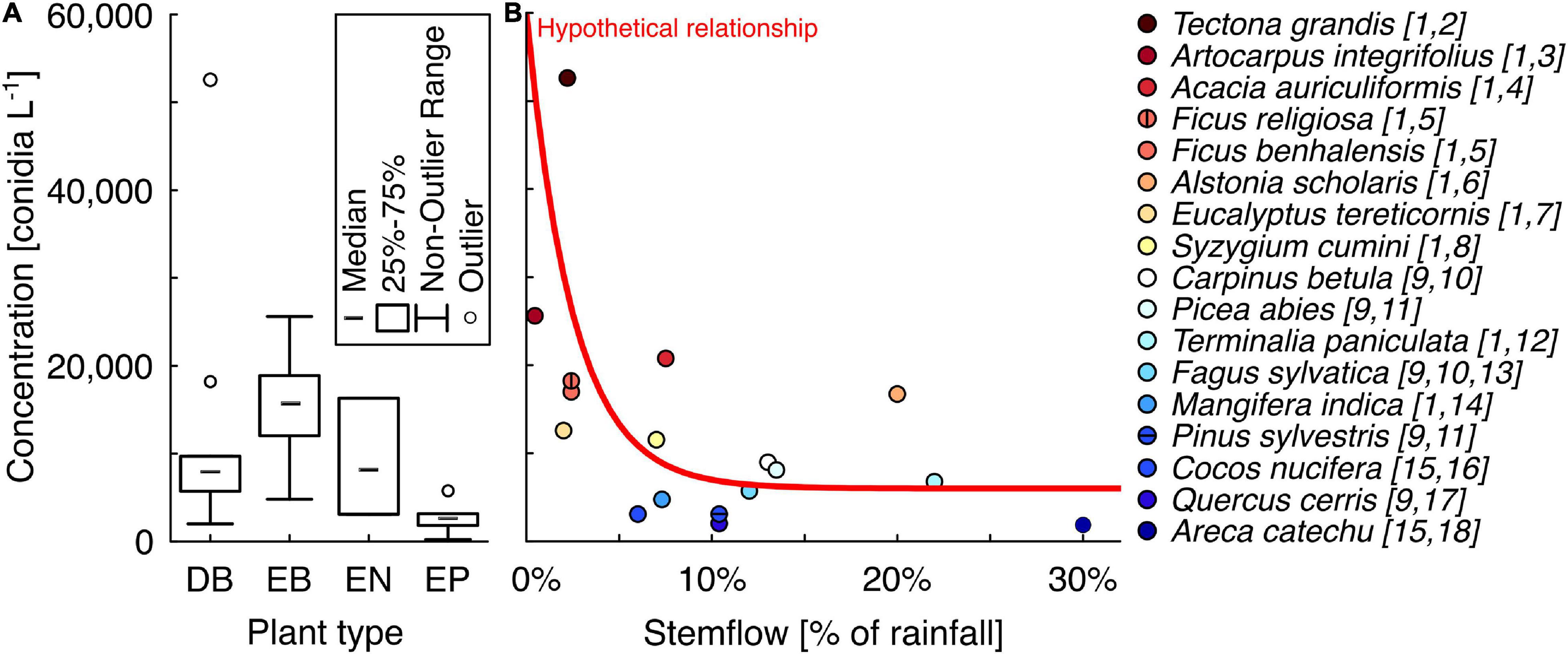
Figure 3. (A) Box and whisker plot comparing the stemflow conidia concentrations measured from different plant types studied to-date: DB, deciduous broadleaved; EB, evergreen broadleaved; EN, evergreen needleleaved; and EP, evergreen palms. (B) Plot of available data on stemflow conidia concentration and the mean stemflow (fraction of rainfall). Note that (i) for many species with observations of conidia concentrations (in panel A) the authors were unable to find published observations of stemflow fraction and (ii) these datasets (conidia concentration and mean stemflow fraction) were collected using different sampling methods (see methods discussion). However, a relationship between these variables, similar to what has been observed for solute concentrations in past work, may be hypothesized. All data for these plots were from publications: [1] Sridhar and Karamchand (2009), [2] Tanaka et al. (2017), [3] Mali et al. (2020), [4] Bruijnzeel et al. (1987), [5] Ficus benjamina from Guevara-Escobar et al. (2007), [6] Ray (1970), [7] Nizinski et al. (2011), [8] Sahu et al. (2006), [9] Gönczöl and Révay (2006), [10] Mitscherlich (1981), [11] Cape et al. (1991), [12] Ndakara (2012), [13] Peck (2004), [14] Ndakara (2016), [15] Ghate and Sridhar [2015c], [16] Serrano (1982), [17] Mosello et al. (2002), and [18] Cheng et al. (2008).
The median of stemflow conidia concentrations reported to date was highest for evergreen broadleaved trees, 15,750 conidia L–1, followed relatively closed by both evergreen needleleaved trees and deciduous broadleaved trees, 8,134 versus 8,000 conidia L–1, respectively, and was lowest for the palms, 2,665 conidia L–1 (Figure 3A). One of the two outliers from the deciduous broadleaved trees was discussed earlier (T. grandis); the second was A. integrifolia (i.e., Artocarpus heterophyllus; Figure 3A). When available conidia concentration data for plant species are plotted against their stemflow fraction, a general trend emerges where stemflow fraction is inversely related to stemflow conidia concentration (Figure 3B). The mechanism behind the hypothetical exponential decay in Figure 3B may be related to greater stemflow resulting in the scouring of fungal conidia from bark surfaces and subsequent dilution. Thus, the scouring by high stemflow rates could exhaust the bark conidia available to stemflow and, thus, greater stemflow production would diminish the total conidia per unit volume. This is analogous to the well-known “first flush dynamics” in watersheds (Sansalone and Cristina, 2004).
Conidia fluxes from tree species in Sridhar and Karamchand (2009) were recently estimated, ranging from 4–278 × 109 conidia ha–1 year–1 (Van Stan et al., 2021). Although conidia concentrations in the stemflow of palms were very low (Figure 3A), they appear capable of generating fluxes of similar magnitude to trees. This may be a function of the palm species typically having greater stemflow fractions than tree species (Serrano, 1982; Frangi and Lugo, 1985; Cheng et al., 2008; Germer et al., 2010). Stemflow fractions have been reported for two of the palm species investigated by Ghate and Sridhar (2015c): 30 and 6% of annual rainfall for A. catechu and C. nucifera, respectively, (Serrano, 1982; Cheng et al., 2008). Given these stemflow fractions (of the 3,780 mm year–1 mean annual rainfall in Mangalore, India) and assuming the published conidia concentrations are representative, then a hectare of C. nucifera or A. catechu would theoretically be able to input 7 × 109 and 26 × 109 conidia ha–1 year–1, respectively, to the soils near their stems.
Conidia Species Composition in Stemflow
Observations and Hypotheses on the Number and Morphology of Conidia Species
The literature synthesis and new data represent observations for 228 fungal species’ conidia in stemflow collected from 58 tree species, 6 palm species, and 1 bamboo species (see dataset, Supplementary Table 1). Conidia observed in all canopy habitats included in this study (stemflow, bark and honeydew) consisted of 368 different fungal species (Supplementary Table 1). When compared to the bark conidia assemblages synthesized from 63 tree species and 3 vine species, there was a significant portion of overlap between stemflow and bark observations (44%, or 102 shared species), suggesting that stemflow rivulets scour conidia from the bark surfaces over which they drain. Fewer shared species were found for stemflow and honeydews (25%, or 56 shared species). Observing fewer shared conidia species between stemflow and honeydew compared to stemflow and bark is reasonable as the honeydews are seasonal and may not cover all the bark area over which stemflow can drain. Half the conidia species observed in stemflow had not been observed in bark or honeydews (114 spp.); these may originate from bioaerosols, the overflow of tree holes, or any other of the myriad microenvironments within canopies (Sridhar, 2009; Magyar et al., 2016b). Thus, these data suggest that stemflow integrates a diversity of canopy microhabitats for conidia as it drains to the surface.
Stemflow conidia have been observed from taxonomically and ecologically heterogeneous groups of fungi (Supplementary Table 1). Several morphologically distinct conidia have been observed in stemflow— many of them have not been identified even to the generic level (Gönczöl and Révay, 2003, 2004, 2006), which confirms the existence of many unknown species in tree canopies. The larger trees seemed to have higher diversity of spores in stemflow, possibly owing to the increased surface area for colonization of bark fissures or the presence of lichens (MacKinnon, 1982). Data available to date indicate that not all tree species are appropriate to develop a high diversity of fungi adapted to stemflow dispersal (Figure 4). For example, the number of conidia species observed in the stemflow of tree species that generate relatively high stemflow fractions (>5% of rainfall across their canopy)— like F. sylvatica, Populus tremuloides, or C. betulus— typically exceed 30 spp. (MacKinnon, 1982; Gönczöl and Révay, 2006; Magyar, 2007). On the other hand, tree species which generate relatively low stemflow fractions (<1% of rainfall across their canopy)—like T. grandis, G. dioica and E. tereticornis— are reported to have only 2–20 spp. (Magyar, 2007; Sridhar and Karamchand, 2009; Supplementary Table 1). An example comparison of selected tree species with published data on bark and stemflow conidia spp., stemflow fraction, and the bark water storage capacity permits further discussion (Figure 4). The more structurally complex, exfoliating bark (E. angustifolia) contains more conidia spp. (n = 121), likely because more types of conidia are trapped by the bark and the spongey structure adsorbs and stores water for a long time, allowing the development of thriving fungal colonies (Magyar, 2008). In addition, the trunk structure of this studied E. angustifolia tree (being tortuous and steeply inclined) is somewhat unsuitable for trickling of rivulets (Pypker et al., 2011). Likely as a consequence of these features, stemflow from E. angustifolia contains the least number of conidia spp. (n = 20) compared to the others, despite its portion of species shared with the bark assemblage is highest (85%). In contrast, beech (F. sylvatica) is typically a tall and straight tree. Stemflow from beech trees’ comparatively simple bark surface structure not only has the most conidia species, but has a greater number of species than observed on the bark surface (n = 35 spp. in stemflow versus 13 spp. on the bark) and only 29% of the bark conidia species were observed in stemflow. Smooth bark trees with low bark water storage capacities generate more stemflow (André et al., 2008; Figure 4), which may wash more bark area clean, integrating a greater amount of canopy microenvironments compared to trees with more complex bark.
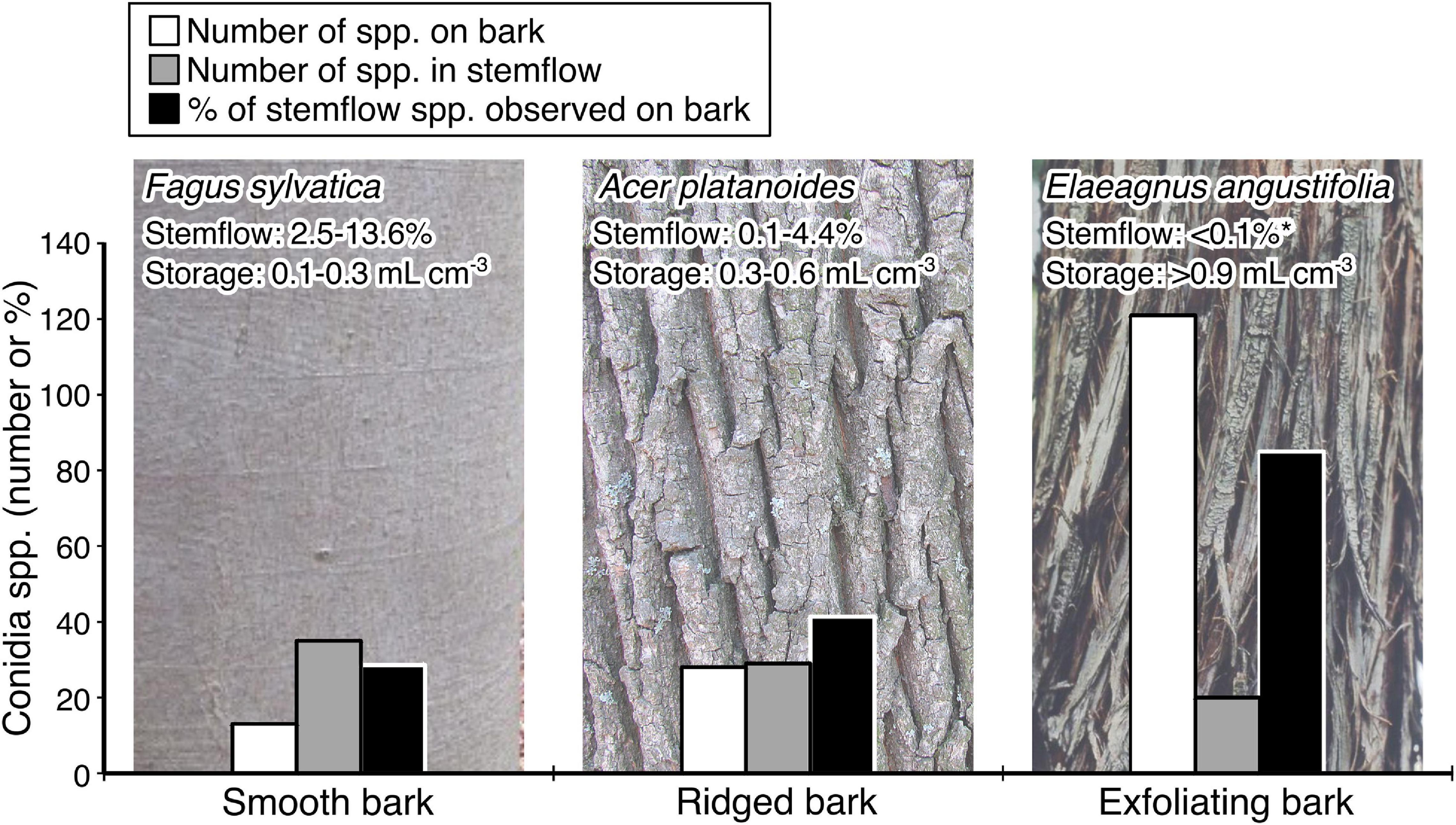
Figure 4. A case study comparison of the number of species of conidia observed in bark (white bar) and stemflow (gray bar) assemblages between species with increasingly complex bark structure. Note that the portion of stemflow conidia species represented by species observed from bark (black bar) samples increases with bark complexity. Conidia data are from published studies (Gönczöl and Révay, 2006; Magyar, 2007, 2008; Révay and Gönczöl, 2011b). Include citations for stemflow and storage estimates. For context, observations of relative stemflow and normative bark water storage capacity are provided: Fagus grandifolia (Giacomin and Trucchi, 1992; Krämer and Hölscher, 2009; Van Stan et al., 2016); Acer platanoides (Valová and Bieleszová, 2008; Schooling and Carlyle-Moses, 2015; Campellone, 2018); Elaeagnus angustifolia (Schooling and Carlyle-Moses, 2015). *Stemflow from E. angustifolia reaching this value only occurred for storms ≥ 10 mm event-1 (Schooling and Carlyle-Moses, 2015). Photographs are all under creative commons licenses: F. sylvatica (Elsner, 2012), A. platanoides bark (Gmihail, 2014), and E. angustifolia (T. Davis Sydnor, https://Bugwood.org).
Regarding the conidia species found in stemflow, mycological studies on stemflow have focused on morphological species identification, specifically for staurosporous (i.e., radiate or branched), scolecosporous (elongate or thread-like), and helicosporous (spiral-like) taxa. It has long been noted that stauro- and scolecosporous conidia are often water-transported (Ingold, 1966). Later findings suggest that conidia of these fungi are produced, liberated and dispersed synchronously with rainfall events (MacKinnon, 1982). Thus, the transport of stemflow-specific fungi initiates by the liberation of conidia from their colonies. The complex shape of their branched conidia is advantageous for dispersal as it might be easily torn off from their conidiophores by water tension than a conidium with a smaller surface area. The density of aquatic hyphomycete spores being ∼500 femtogram μm–3 (Findlay and Arsuffi, 1989; Bärlocher, 2020), spore dispersal is then followed by a mostly passive drift in the draining stemflow. Additionally, a branched spore occupying several planes can be refloated more easily after settling and moved more freely on water films (MacKinnon, 1982).
Fungal genera on above ground substrates have been found to more likely produce allantoid (curved elongated) spores, to lower the risk of precipitation-related wash out (Calhim et al., 2018). Thus, we note that safe arrival on specific substrates is arguably another important driver of spore morphological evolution in addition to dispersal (Calhim et al., 2018). Still, the branched form of conidia is the product of convergent evolution and secondary adaptation to aquatic mode of life (Ingold, 1975; Belliveau and Bärlocher, 2005; Sudheep and Sridhar, 2010). Ingold (1942, 1953) suggested three selective pressures responsible for branched shapes of conidia: (a) delayed sedimentation for dispersal, (b) settlement on a suitable substrate, and (c) prevention from ingestion by invertebrates. Such conidia are also thought to hold water around the conidium, thereby increasing the possibility of quick germination (Sridhar and Karamchand, 2009). The following subsections will discuss the two different subgroups of these branched, elongated or twisted conidia: “true” aquatic (or Ingoldian) hyphomycetes and a paraphyletic group of conidia currently un-named, which we propose to call “dendronatant fungi” (as discussed in section “Observations and hypotheses on non-Ingoldian, ‘dendronatant fungi’ in stemflow”).
Observations and Hypotheses on Ingoldian Hyphomycetes in Stemflow
The Ingoldian fungi are well-known from streams, but their discovery in stemflow was highly surprising. They are reported from stemflow in both temperate and tropical areas (e.g., Sridhar and Karamchand, 2009; Révay and Gönczöl, 2010). They have been early and regularly reported to occur in a variety of environments other than their preferred habitat. Of all the fungal species identified in the studies synthesized here, 19% (n = 70) of the species are considered Ingoldian fungi (Supplementary Table 1). When monitoring stemflow, Bandoni (1981) observed spores of Gyoerffyella biappendiculata, a species he considered as an Ingoldian fungus. Later on, the conidia of many other Ingoldian hyphomycetes typical in temperate streams are reported to be common in stemflow and throughfall samples (e.g., Anguillospora crassa, A. longissima, Alatospora, Articulospora, Flagellospora curvula, Tricladium spp., and Varicosporium spp.; Chauvet et al., 2016). It was also shown that some Ingoldian fungi can actively grow and sporulate in terrestrial litter and soil (Sridhar and Bärlocher, 1993; Sridhar et al., 2020).
Although these fungi have been reported from the phyllosphere of 57 plant species (Chauvet et al., 2016), the growth and sporulation of Ingoldian fungi in tree canopies is only indirectly known by the presence of their conidia in stemflow (Czeczuga and Orłowska, 1999; Sridhar and Karamchand, 2009). It was postulated that there is the existence of a guild of fungi that may “function in canopies much as classical Ingoldian aquatic hyphomycetes in streams” (Carroll, 1981). In addition, a gradient (or zonation) may develop across the canopy owing to stable and unstable niches with macro- and micro-niches (i.e., for tree holes or complex bark structures). Still, many questions exist regarding the ecology of these fungi: Can Ingoldian hyphomycetes adapt to sporulation in free water in canopies? How did these fungi, well-known inhabitants of streams, “climb up” to colonize tree tops? These are amongst the curious open questions in fungal ecology. There are many speculations and hypotheses we may synthesize here (Figure 5). Selosse et al. (2008) hypothesized that large numbers of conidia in air bubbles of stream foam may be dispersed through wind or aerosols and onto tree canopies (Figure 5a). However, these conidia were almost absent during the air monitoring of spores of two decades (Magyar, unpublished) and were not detected in air samples. Therefore, the hypothesis of airborne dispersal of Ingoldian conidia in large numbers seems to be implausible. Another common mechanism is spore dispersal by rain splash, which is widely known in some plant pathogenic fungi (Figure 5b). It occurs when a rain drop falls onto a surface covered by a thin film of water. By this impact, many (100–5,000) secondary droplets produced at its periphery (Madden, 1992). Minute secondary droplets are observed to be blown away by strong wind from stemflow dripping from bark extremities (Magyar, personal field observations, Figure 5c). Other types of dispersal mechanisms prevalent during rainfall may also considered, e.g., wet shake-off (Figure 5d). It is possible to speculate that some spores transported by stemflow may be aerosolized by secondary splash droplets, stemflow dripping or wet-shake off (Figures 5b,c2,d) and transported to longer distances by wind (Figure 5f), but further studies dealing with the comparison of spore content of splash or stemflow or fog samples are necessary to confirm this view. Some branched spores are observed on the feet of birds, which may be transported to the tree tops (Vass, 2015; Figure 5e). Insects have also been observed drinking regularly from the foam floating on a creek, where Ingoldian fungi are common. It is possibly a way to transport these conidia to the trees too (Figures 5g, 6a). Rainwater containing spores of Ingoldian spores were found to be accumulated in walnut shells. A network of micro-telmata (walnut shells, snail sells, and spots etc.) between trees and a creek may be contribute to a horizontal mesoscale spore dispersal by rainsplash and throughfall (Figures 5m, 6b). Similarly, a network of dendotelmata were found in Õrség, Hungary on Alnus and Carpinus trees, with many holes per each tree at different heights (Figure 6c). Hypothetically such splash from these holes can provide the vertical mesoscale transport of spores, too (Figure 5n). However, the above-mentioned transport mechanisms seem to be episodic and unlikely to account for the abundance and diversity of these fungi in stemflow. The most likely explanation involves fungal sexual reproductive structures (teleomorphs) which have non-branched spores adapted to wind dispersal (Figure 5f1–3) unlike branched ones (Figure 5f4). For example, studies on anamorph-teleomorph connections of Ingoldian hyphomycetes showed that the majority of species have evolved from ascomycetes in decaying tree branches in streams (Ranzoni, 1956; Webster and Descals, 1979, Marvanová, 1997; Sivichai and Jones, 2003). To date, however, this remains an untested hypothesis. To test this hypothesis, studies must collect air samples to ascertain the presence of non-branched spores of teleomorphs by a combination of microscopical and molecular techniques.
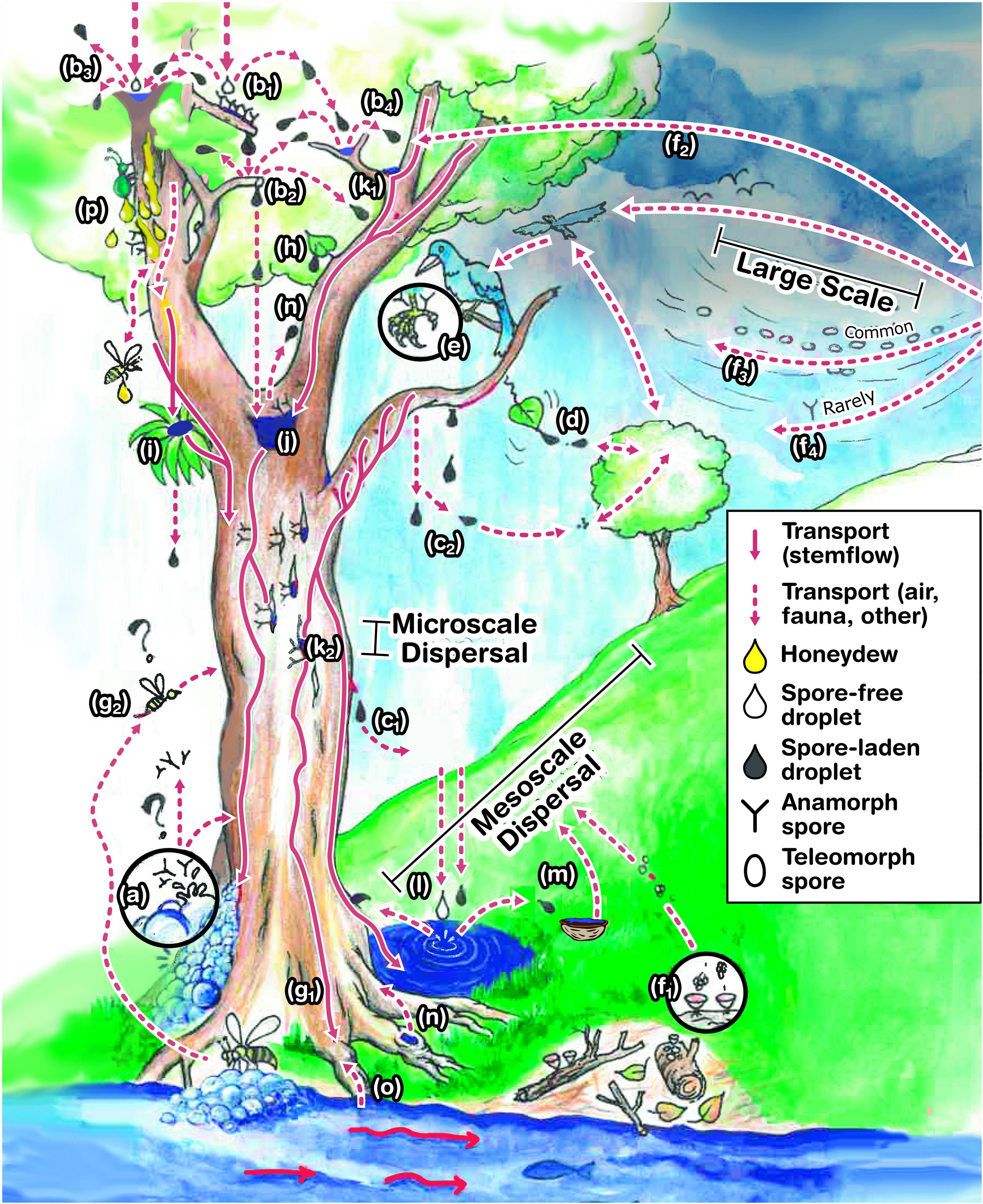
Figure 5. Illustration synthesizing the hypothesized source, fate and transport of Ingoldian and dendronatant conidia found in stemflow, including: (a) bubbles; (b) splash; (b1) spore-free rain droplet hits a fungal colony; (b2) a spore-laden drop accumulated at the end of twig; (b3) spore-free rain droplet hits spore-laden water film; (b4) spore-laden droplet hits spore-laden water film; (c) aerosolized stemflow from (c1) drips from branches and (c2) bark extremities; (d) shaking release and dispersal; (e) epi-faunal exchange; (f) wind dispersal of teleomorph spores (f1) from local or (f2–3) distant sources; and (f4) while airborne dispersal of anamorphs seems to be rare; (g1,2) exchange by invertebrates visiting streams; phytothelmata in epiphytes; (h) throughfall; overflow from (j) treeholes, (k) aquatic microhabitats in (k1) branch junctions and (k2) bark fissures (note that some is filled with rainwaters while others are out of the way of rivulets and consequently remains dry, some of them of low fungal growth; (l) rain and throughfall generates splash transfer between tree, stream and nearby puddles, (m) micro-thelmata (e.g., walnut shells) and (n) treeholes; (o) endophytic colonization and dispersal; and (p) spore capture and transport due to honeydew flow and attracted invertebrates.
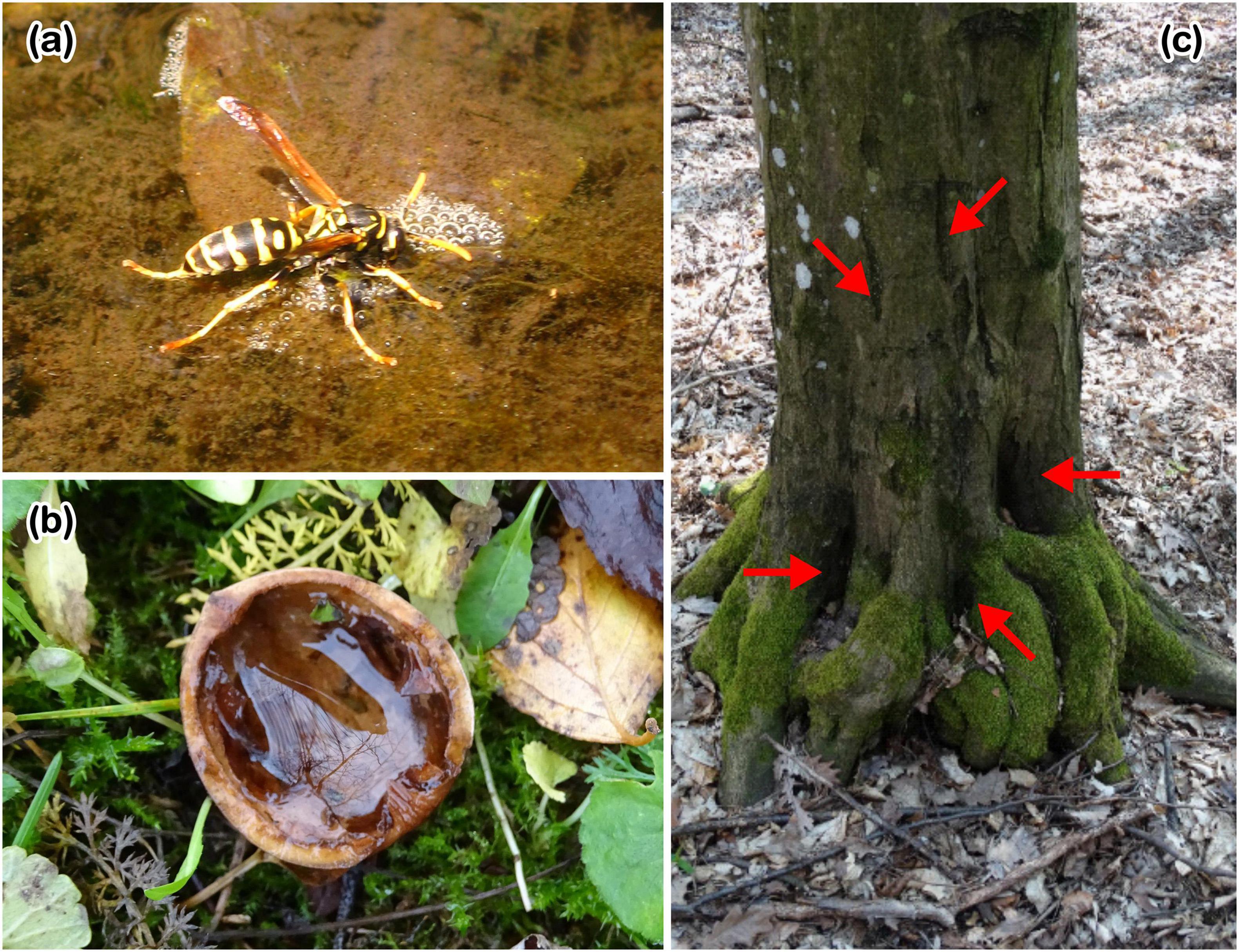
Figure 6. Photographs of potential vectors and microhabitats that may act as sources (or sinks) for stemflow conidia. (a) an insect drinking from the foam floating on a creek, where Ingoldian fungi are common. It is possibly a way to transport these conidia from streams to the tree canopy. (b) Rainwater accumulated in a walnut shell (i.e., a “micro-phytothelma”) between trees and a creek, containing spores of Ingoldian and dendronatant fungi. It may enable a mesoscale spore dispersal by rainsplash and throughfall between a network of such telmata (nutshells, pots, and snail shells, etc.). (c) Red arrows point to a network of dendrotelmata found in Õrség, Hungary on Alnus and Carpinus trees, which can hypothetically provide vertical mesoscale transport of spores (Credit for all photos: D. Magyar).
Sudheep and Sridhar (2010) suggest that the life cycle of these fungi alternates between aquatic and canopy habitats. Tropical areas, like the south-west coast of India, receives substantial rains during monsoon. Thus, occurrence of Ingoldian fungi in tree canopies is not surprising in these regions (Sridhar, 2009). One can speculate that the great quantity of rainfall in the monsoon season may create continuous aquatic habitats in canopies. Bandoni (1981) suspected that the conidia of fungi formed in tree canopies were directly transported to streams through stemflow, throughfall, or invertebrates (Figure 5g). Some evidence also suggests that Ingoldian hyphomycetes survive under terrestrial conditions due to their teleomorph states (Chauvet et al., 2016). It is also known that Ingoldian hyphomycetes can survive several of the environmental stresses likely experienced in tree canopies (e.g., pollution or water intermittency; Vass et al., 2013; Ghate and Sridhar, 2015b). Since these fungi are also common in trees of urban environments of polluted areas, further studies should be aimed to study the environmental role of these fungi in such habitats.
Observations and Hypotheses on Non-Ingoldian, “Dendronatant Fungi” in Stemflow
In our data synthesis (including new data), 19% of the total spore species are considered to be Ingoldian fungi (Supplementary Table 1). Stemflow is rich in numerous other, morphologically complex conidia that have not been connected to Ingoldian fungi (20% of the total spore species). These spores are hyaline/subhyaline stauro- and scolecospores (and some elongated phragmospores), apparently adapted to dispersal in stemflow. After their discovery, these conidia from non-Ingoldian, canopy-derived fungi were labeled with tentative names like “arboreal aquatic hyphomycetes” (Carroll, 1981) or “terrestrial aquatic hyphomycetes” (Ando, 1992), but these names appear to be inadequate (see Gönczöl and Révay, 2006). Another name, “canopy fungi” were also used for this group, however, this name, too, can be misleading as it may be thought to include foliar fungi non-adapted to stemflow dispersal, like conidia of powdery mildews and smuts. In the following parts, we refer to these fungi as a new paraphyletic group, using a name derived from the Greek “dendro” (for tree) and Latin “natant” (for swimming): “dendronatant fungi.” Many studies have reported the diversity and worldwide presence of conidia from dendronatant fungi in stemflow (MacKinnon, 1982; Ando and Tubaki, 1984a, b; Tubaki et al., 1985; Gönczöl and Révay, 2003, 2004, 2006; Magyar et al., 2005, 2016b; Sridhar et al., 2006; Karamchand and Sridhar, 2008; Sridhar, 2009; Sridhar and Karamchand, 2009; Révay and Gönczöl, 2010, 2011a,b; Sudheep and Sridhar, 2010). Note that some morphologically complex conidia that are not associated with dendronatant fungi have also been reported in stemflow (and bark), like helicosporous fungi, the most common of which include the Helicomyces anamorph of Tubeufia palmarum, Helicomyces and Helicosporium spp.
An intensive search for the source (i.e., sporulating colonies and habitats) of these dendronatant fungi, has resulted in the description of new species, while many others are still unknown, even in metropolitan environment (e.g., Sokolski et al., 2006; Magyar and Révay, 2008, 2009a,b; Magyar et al., 2017b,2018). The ecological role and the source of the conidia of these fungi remain incomplete (Révay and Gönczöl, 2010). Some of them seem to live endophytically in various plant tissues, for example, Dwayaangam colodena was proved to be an endophyte in canopy needles of black spruce (Picea mariana) needles (Sokolski et al., 2006; Figure 5o). Others may live in association with epiphytic ferns, bryophytes and lichens. For example, Sridhar et al. (2006) suggested that water-borne hyphomycetes exist in rhizomes of ferns as endophytes in tropical regions (Figure 5i). The majority of these dendronatant fungi species are probably saprotrophs inhabiting senescent or dead leaves trapped in the canopies, where tree holes (Figure 5j), junctions of branches (Figure 5k1), and fissures of rough cortex of trunks (Figure 5k2) serve as ephemeral aqueous microhabitats for these fungi (Magyar, 2008; Magyar et al., 2017b). These accumulation areas support many saprotrophic invertebrates on microliter and canopy soil, their parasites have also appeared here, like predacious fungi of amoebae, nematodes and rotifers. Dwayaangam heterosporais known to parasitize eggs of rotifers and nematodes (Barron, 1991). Lecophagus vermicola hunts nematodes applying an unusual strategy (Magyar et al., 2016a). Specifically, the fungus captures its nematode victims with adhesive knobs and colonizes its prey with a mycelium of rather broad hyphae on which, again, adhesive knobs are formed which penetrate the nematode’s cuticule. As colonized nematodes form a cluster, they become a network enabling the capture of more prey. The fungus lives in the ephemerally aquatic habitat of bark fissures. Stemflow also provides water to the growth of bark-inhabiting fungi colonizing deeper areas of bark fissures, where their spores are present in large number (Magyar, 2008). Camposporium cambrense and C. ontariense are reported to grow and sporulate heavily on the bark cortex. Arxiella terrestris, C. japonicum, C. pellucidum, Diplocladiella scalaroides, Endophragmiella taxi, Excipularia fusispora, Oncopodiella, and Triadelphia spp. are primarily known as wood or leaf litter inhabiting fungi may also live on and derived from dead parts of the live trees (Gönczöl and Révay, 2004). It was suggested that many litter inhabiting fungi may colonize their substrates earlier than when the leaves reach the ground (Gönczöl and Révay, 2004).
Massive deposits of pollen grains and spores are found in bark fissures. Consequently, pollen and spore (or myco-) parasitic fungi sporulate here and rely on stemflow-transported spores to colonize new bark fissures. Branched conidia of pollen parasitising fungi (e.g., Mycoceros and Retiarius spp.) show an adaptation not only for dispersal in stemflow but also trapping pollen grains with specialized arms (Magyar et al., 2018). Most of these dendronatant fungi are little known and hardly studied owing to their sporulating colonies being hidden in bark fissures. Their colonies are tiny, being hardly visible even with the high magnification of stereomicroscopes (Magyar et al., 2018) and lack conidiophores or can be conspicuously micronematous (Ando and Kawamoto, 1986; Ando, 1992). Often identification is difficult or impossible with isolates in pure culture that fail to produce spores or identifiable structures. A special sampling method using adhesive, pressure-sensitive acrylic strips allows observation of sporulation and substrate preference (Magyar and Révay, 2009b) and has led to the discovery of a new habitat in accumulation areas of bark fissures (Magyar, 2008). Similarly, insertion of latex-smeared slides in the canopy junctions may also trap conidia flowing down the stem (Ghate and Sridhar, 2015a). For species that do not sporulate on artificial media, a method of DNA extraction from single conidia was developed as an alternative to perform phylogenetic research (Magyar et al., 2016a).
Finally, these dendronatant fungi seem to be adapted to this habitat, especially in microscale dispersal. Such spores are observed to reach and colonize new accumulation areas, i.e., another bark fissure on the host tree (Magyar, 2008). Since these fissures are found downstream of stemflow, spores tend to have shapes which allow anchoring (Figure 7). The K- or Y-shaped species (e.g., Trinacrium and Retiarius) or multiple, long arms (e.g., Mycoceros, Dwayaangam) seem to be a common, effective morphological feature to enable the anchoring of conidia carried by stemflow onto substrates (Figure 7)—which also may serve as food for the fungus (commonly microlitter, pollen or other fungi). Another adaptation of stemflow dispersal seems to be the development of protruding hyaline cells, or horns, on pigmented, multi-celled spores (Excipularia, Oncopodium and Oncopodiella spp., and Rebentischia unicaudata; Magyar and Révay, 2009b). Dendronatant fungi appear to have analogous or convergent evolutions as the Ingoldian hyphomycetes of streams, because they experience the same problem in running waters: spores are non-motile, and passive transport is dominated by rainwater flowing toward the soil (Chauvet et al., 2016). Colonization of stationary substrates in streams (litter and wood), and in stemflow (e.g., microlitter in accumulation areas) may help some species to overcome the risks of total removal and extinction due to unidirectional flow of water.
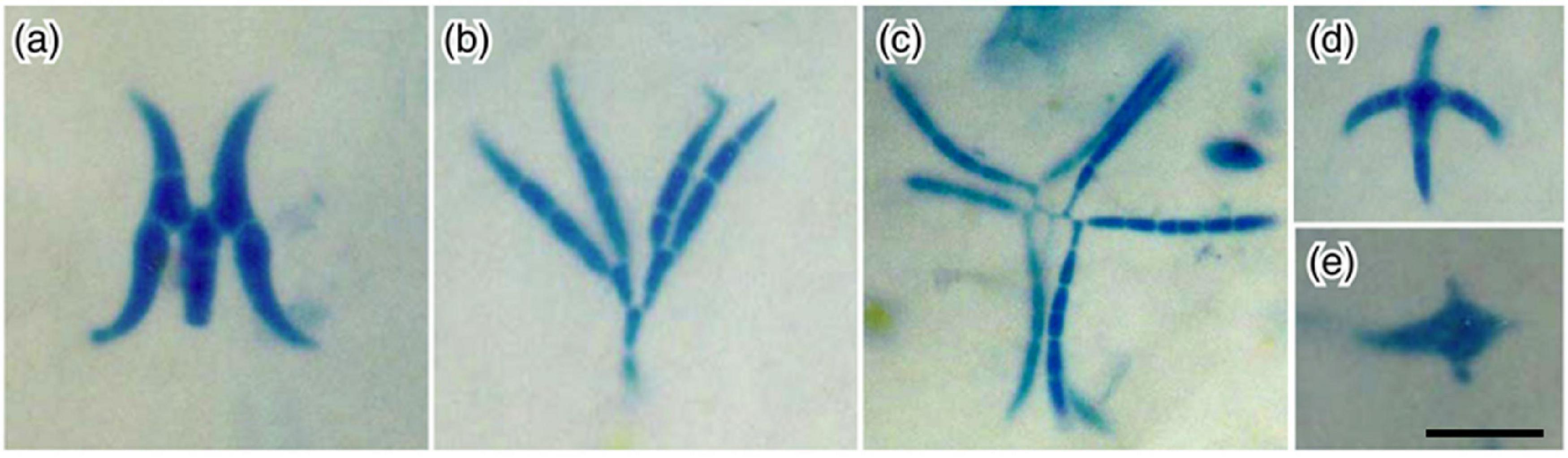
Figure 7. Example conidia of water-borne hyphomycetes recovered from stemflow samples: (a) Dwayangam cornuta; (b) D. dichotoma; (c) Dwayangam sp.; (d) Lemonniera cornuta; (e) Tumularia tuberculata collected in India (credit: K. Sridhar). Scale bar = 20 μm.
Stemflow’s Hypothetical Impact on Bark Fungal Community Patterns
Stemflow must interact with bark microhabitats as it drains to the surface and, as a result, may not only transport conidia, but may hypothetically affect the bark reservoir’s fungal community composition. Patterns of bark microhabitats and stemflow-related water processes hypothetically align (Figure 8a). Stemflow rivulets drain along the bark microrelief, tending to follow furrows (Tucker et al., 2020), especially if the bark is not particularly spongey (Brown and Barker, 1970; Van Stan and Levia, 2010). Stemflow water that saturates bark furrows may be better sheltered from evaporative drivers, like wind and radiation, than waters draining along bark ridges (Figure 8a), allowing bark moisture to persist for longer periods of time in bark furrows than on ridges (Young, 1937). These bark microhabitats collect substantial particulates, including conidia, as several hundreds of kilograms of dust per year may be captured from a canopy capable of scavenging 66–80% of atmospheric aerosols (Steubing and Kirschbaum, 1976). The total amount of dust (PM2.5) removed annually by trees is enormous, varied from 4.7 tonnes in Syracuse (NY, United States) to 64.5 tonnes in Atlanta (GA, United States; Nowak et al., 2013).
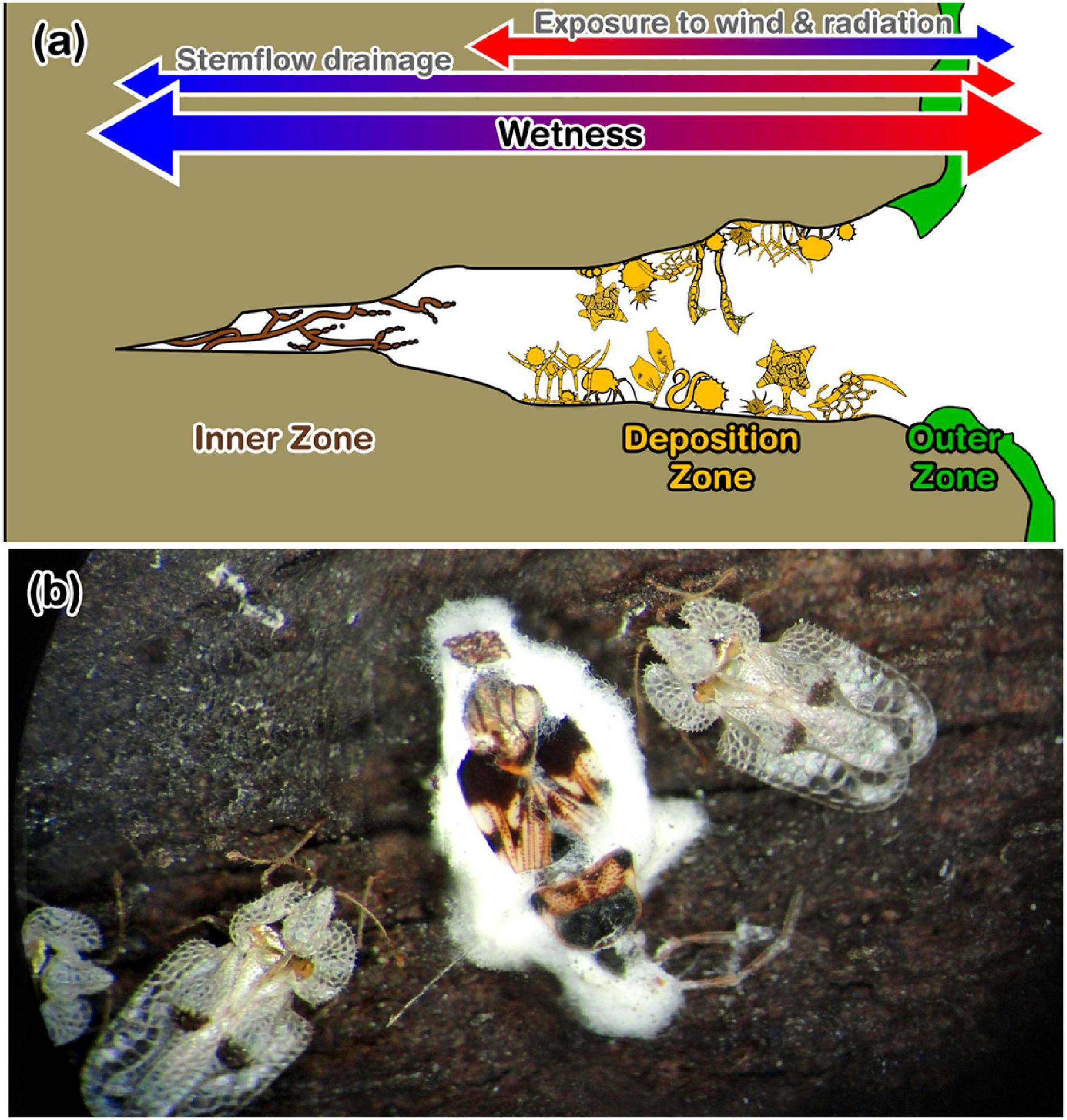
Figure 8. (a) Zones of the bark microhabitat per Magyar (2008), their relative wetness (per stemflow and atmospheric exposure), and their observed microhabitat components. The outer zone consists of lichens, mossed and melanized fungal mycelia. Components of the deposition zone are diverse and abundant, like spores, pollen grains, dust, mites, nematodes and testate amoebae. The inner zone primarily hosted dematiaceous (or “black”) fungi colonies. (b) Photograph showing example fungal infection of overwintering insects by bark fungi: Beauveria sp. on Heteroptera sp. and Corythucha ciliata on a Platanus tree in Budapest, Hungary (credit: D. Magyar).
There are many anemophilous fungal species (Ingold, 1971) which may be deposited on bark surfaces, whereafter their survival and reproduction may depend on microclimatological conditions, especially moisture conditions (Chauvet et al., 2016). Evidence that many scavenged aerosol fungi are unable to thrive on bark surfaces can be found through comparisons of conidia assemblages simultaneously collected from air and bark samples, which differ markedly (Magyar, 2008). In fact, common airborne fungi are rarely seen in stemflow (or throughfall; Gönczöl and Révay, 2004, 2006; Magyar et al., 2005). Thus, fungi community patterns throughout the bark microhabitat may be significantly influenced by interactions between the bark microclimate and its major moisture source, stemflow.
Along this hypothetical bark moisture gradient (Figure 8a), microhabitats have been previously described by Magyar (2008), where lichens, mosses and melanized fungal mycelia dominate the “outer zone” on the ridge, which transitions into a “deposition zone” hosting a large variety of captured aerosol particulates (including aerially-transported conidia and pollen) and small metazoans, until reaching an “inner zone” at the base of bark furrows dominated by large “black” (i.e., dematiaceous) fungi colonies (Figure 8a). Many of the fungal community members that reside among these bark microhabitats are rarely observed, as they cannot be detected using standard “washing and plating” techniques; however, understanding their ecological roles and environmental controls may yield insights into these communities’ function. For example, are they pathogenic or do they support a healthy bark microbiome? Do fungal community members of the bark microbiome control invertebrate pests? Fungal epidemics of overwintering insects and nematodes by bark fungi are often observed (Lecophagus, Dactylaria, Dactylellina, and Beauveria spp.; e.g., Figure 8b). Are deep, moist bark fissure microhabitats shelters for many fungal species, enabling survival during unfavorable conditions? Theoretically, stemflow not only has multiple fungal microhabitats with which it may interact, but stemflow dynamics themselves may influence bark fungal communities and their patterns.
Conclusion
Hypotheses Based on Available Data and Theory
The following hypotheses regarding stemflow conidia assemblages emerged from our analysis of published observations to date:
H1. Stemflow conidia concentrations are inversely related to the amount of rainfall that a tree drains as stemflow. This suggests that stemflow conidia concentrations are limited by the conidia “reservoir” on the bark surfaces over which stemflow drains. Future work may be merited on quantifying the size and dynamics of the bark conidia reservoir.
H2. Stemflow transports an ecologically and biogeochemically relevant amount of conidia (>109 ha–1 y–1) to the localized soil areas at the base of individual trees. This suggests that stemflow conidia fluxes may represent “hot spots and moments” of fungal dispersal to litter, soil, and potentially root areas. Stemflow infiltration areas range from 10–1–101 m2 tree–1 (Van Stan and Allen, 2020) and stemflow pulses have been found to influence litter layers (Tanaka et al., 1991; Iida et al., 2005; Rashid and Askari, 2014) and drain throughout habitats of the plant microbiome, including the rhizosphere and pedosphere (Van Stan et al., 2020b).
H3. Diversity of stemflow conidia assemblages increases with increasing stemflow generation. Available observations suggest that tree canopies which generally produce large amounts of stemflow, may host a greater diversity of fungal species adapted to stemflow dispersal. Such high conidial diversity in stemflow, however, may be time-dependent, as concentrations tend to be higher at the early minutes of the rain event for canopies with low flow resistance.
H4. Origins of conidia from Ingoldian and dendronatant fungi in stemflow can vary depending on multiple site-specific, species-specific and/or canopy structural properties, e.g.: (i) presence of aerosolized teleomorphs (Ranzoni, 1956; Webster and Descals, 1979, Marvanová, 1997; Sivichai and Jones, 2003), (ii) endophytes of leaves or epiphytes (Sokolski et al., 2006; Sridhar et al., 2006), (iii) birds that interact with marine and canopy environments (e.g., Vass, 2015), and (iv) saprotrophs inhabiting senescent or dead leaves trapped in the canopies (Magyar, 2008; Magyar et al., 2017a).
H5. Many dendronatant fungal species found in stemflow appear to be adapted to stemflow dispersal. Observations suggest that conidia of some species are produced, liberated and dispersed synchronously with rainfall events (MacKinnon, 1982). Some conidia also have unique structures (for example: K- and Y-forms; multiple long arms; protruding horn-like hyaline cells) that assist in film (i.e., stemflow) dispersal and anchoring in other canopy habitats (Bandoni and Koske, 1974; Magyar and Révay, 2009b; Chauvet et al., 2016).
H6. Stemflow-bark interactions may influence patterns in bark fungal community structure. This is based on comparing patterns of bark microhabitats (Magyar, 2008) and stemflow-related moisture/microclimatological processes (Tucker et al., 2020), which hypothetically align.
We reiterate that these are hypotheses based on the data available to date and remain to be tested. The available data underlying these hypotheses have several caveats (discussed throughout the preceding sections); however, we hope that these hypotheses will provide a framework for future work at the intersection of mycology and critical zone science in forests, especially during storms.
Frontiers at the Intersection of Bark, Stemflow and Fungi
Surprisingly, trees showing high fungal diversity were found in urban environments. It can be hypothesized that the canopy of urban trees are rich in different pollutants, used as nutrient source of fungi. Organic particles deposited on the canopy are washed off to the fissures, where bark inhabiting fungi digest them. Unlike trees, most fungi are not sensitive to urban stress (especially extremotolerant species). Traffic-related wounds, as well as air and soil pollution are major challenges to urban trees, but it is positive to opportunistic fungal pathogens. Low biodiversity of urban tree lines increases the risk of plant epidemics. As a result of globalization, new pests are introduced frequently. Some invasive insects produce honeydew on urban trees, where sooty molds develop in high quantities. Plant pathogenic fungi on trees, like Schizophyllum commune threaten susceptible tree taxa in the parks and streets (Vulinoviæ et al., 2019). On the other hand, non-pathogenic fungi form a protective layer on their aerial surfaces. Mycoparasitic, nematode-destroying and entomopathogenic fungi are common on the bark. A two-step self-cleaning mechanism may be functioning on trees. First, a physical clearing by water-repellent characteristic of leaf cuticle and the covering waxes. Surface particles are picked up by rolling water droplets and are thus easily cleaned off the surface. If a water droplet rolls across a contaminated leaf surface the adhesion between the particle, irrespective of its chemistry, and the droplet is higher than between the particle and the surface. Self-cleaning is the well-known prevention of contamination of the area of the phylloplane exposed to light resulting in reduced photosynthesis (Koch et al., 2008). The second step of self-cleaning is the “digestion” of organic particles by the fungus layer on the large surface area of the bark. It was observed that a mixed vegetation of Pinus, Elaeagnus and Platanus tree species results in the appearance of pollen-consuming microfungi. Pines provide nutrient-rich pollen grains while Elaeagnus and Platanus bark offer suitable physical environment for fungal development (Magyar et al., 2017a). Airborne pollen therefore can may be reduced by planning green areas. Fungal diversity of urban trees may have many other unexplored uses. Stemflow-dispersed spores are also reported to be effective in biological control of insects. Beauveria bassiana is a common entomopathogenic hyphomycete that, when the aqueous suspension of this fungus (as a bioprotectant) sprayed onto trees to form stemflow, the treatment was as effective similar to chemical insecticides (Jakus and Blazenec, 2011). Thus, fundamental research on stemflow and its conidia may not only yield theoretical insights regarding ecohydrological and biogeochemical processes, it may yield practical insights and “myco-solutions” regarding tree health and management.
Data Availability Statement
The original contributions presented in the study are included in the article/Supplementary Material, further inquiries can be directed to the corresponding author/s.
Author Contributions
DM: conceptualization, supervision, writing—original draft preparation, photos, and spore identification. JV: conceptualization, statistical analysis, and writing—original draft preparation. KS: review, photos, and spore identification. All authors contributed to the article and approved the submitted version.
Conflict of Interest
The authors declare that the research was conducted in the absence of any commercial or financial relationships that could be construed as a potential conflict of interest.
Acknowledgments
The authors would like to thank János Gönczöl for his valuable comments.
Supplementary Material
The Supplementary Material for this article can be found online at: https://www.frontiersin.org/articles/10.3389/ffgc.2021.623758/full#supplementary-material
Footnote
References
Akinsoji, A. (1991). Studies on epiphytic flora of a tropical rain forest in southwestern Nigeria: II: bark microflora. Vegetatio 92, 181–185. doi: 10.1007/BF00032605
Ando, K., and Kawamoto, I. (1986). Arborispora, a new genus of hyphomycetes. Trans. Mycol. Soc. Jpn. 27, 119–128.
Ando, K., and Tubaki, K. (1984a). Some undescribed hyphomycetes in the rain drops from intact leaf-surface. Trans. Mycol. Soc. Jpn. 25, 21–37.
Ando, K., and Tubaki, K. (1984b). Some undescribed hyphomycetes in the rain water draining from intact trees. Trans. Mycol. Soc. Jpn. 25, 39–47.
André, F., Jonard, M., and Ponette, Q. (2008). Influence of species and rain event characteristics on stemflow volume in a temperate mixed oak–beech stand. Hydrol. Process. Int. J. 22, 4455–4466. doi: 10.1002/hyp.7048
Aung, K., Jiang, Y., and He, S. Y. (2018). The role of water in plant–microbe interactions. Plant J. 93, 771–780. doi: 10.1111/tpj.13795
Backnäs, S., Laine-Kaulio, H., and Kløve, B. (2012). Phosphorus forms and related soil chemistry in preferential flowpaths and the soil matrix of a forested podzolic till soil profile. Geoderma 189, 50–64. doi: 10.1016/j.geoderma.2012.04.016
Baldrian, P. (2017). Forest microbiome: diversity, complexity and dynamics. FEMS Microbiol. Rev. 41, 109–130.
Bandoni, R. J. (1981). “Aquatic hyphomycetes from terrestrial litter,” in The Fungal Community, Its Organization and Role in The Ecosystem, eds D. T. Wicklow and G. C. Carroll (New York, NY: Marcel Dekker), 693–708.
Bandoni, R. J., and Koske, R. E. (1974). Monolayers and microbial dispersal. Science 183, 1079–1081. doi: 10.1126/science.183.4129.1079
Bärlocher, F. (2020). “Sporulation by aquatic hyphomycetes,” in Methods to Study Litter Decomposition, eds F. Bärlocher, M. Gessner, and M. Graça (Cham: Springer). doi: 10.1007/978-3-030-30515-4
Barron, G. L. (1991). A new species of Dwayaangam parasitic on eggs of rotifers and nematodes. Can. J. Bot. 69, 1402–1406. doi: 10.1139/b91-180
Belliveau, M. J.-R., and Bärlocher, F. (2005). Molecular evidence confirms multiple origins of aquatic hyphomycetes. Mycol. Res. 109, 1407–1417. doi: 10.1017/s0953756205004119
Bittar, T. B., Pound, P., Whitetree, A., Moore, L. D., and Van Stan, J. T. (2018). Estimation of throughfall and stemflow bacterial flux in a subtropical oak-cedar forest. Geophys. Res. Lett. 45, 1410–1418. doi: 10.1002/2017gl075827
Brown, J. H. Jr., and Barker, A. C. Jr. (1970). An analysis of throughfall and stemflow in mixed oak stands. Water Resourc. Res. 6, 316–323. doi: 10.1029/wr006i001p00316
Bruijnzeel, L. A., Sampurno, S. P., and Wiersum, K. F. (1987). Rainfall interception by a young Acacia auriculiformis (A. Cunn) plantation forest in West Java, Indonesia: application of Gash’s analytical model. Hydrol. Process. 1, 309–319. doi: 10.1002/hyp.3360010402
Calhim, S., Halme, P., Petersen, J. H., Læssøe, T., Bässler, C., and Heilmann-Clausen, J. (2018). Fungal spore diversity reflects substrate-specific deposition challenges. Sci. Rep. 8:5356. doi: 10.1038/s41598-018-23292-8
Campellone, S. V. (2018). An Investigation into the Factors Affecting Street Tree Rainfall Interception. Masters Thesis. Philadelphia, PA: Drexel University.
Cape, J. N., Brown, A. H. F., Robertson, S. M. C., Howson, G., and Paterson, I. S. (1991). Interspecies comparisons of throughfall and stemflow at three sites in northern Britain. For. Ecol. Manag. 46, 165–177. doi: 10.1016/0378-1127(91)90229-o
Carpenter, S. R. (1982). Stemflow chemistry: effects on population dynamics of detritivorous mosquitoes in tree-hole ecosystems. Oecologia 53, 1–6. doi: 10.1007/bf00377128
Carroll, G. C. (1981). Mycological Inputs to Ecosystems Analysis. The Fungal Community: Its Organization and Role in the Ecosystem. New York, NY: Marcel Dekker, 557–580.
Chauvet, E., Cornut, J., Sridhar, K. R., Selosse, M. A., and Bärlocher, F. (2016). Beyond the water column: aquatic hyphomycetes outside their preferred habitat. Fungal Ecol. 19, 112–127. doi: 10.1016/j.funeco.2015.05.014
Cheng, J. D., Lin, J. P., Lu, S. Y., Huang, L. S., and Wu, H. L. (2008). Hydrological characteristics of betel nut plantations on slopelands in central Taiwan. Hydrol. Sci. J. 53, 1208–1220. doi: 10.1623/hysj.53.6.1208
Czeczuga, B., and Orłowska, M. (1999). Hyphomycetes in rainwater, melting snow and ice. Acta Mycol. 34, 181–200. doi: 10.5586/am.1999.014
D’Amico, V., and Elkinton, J. S. (1995). Rainfall effects on transmission of gypsy moth (Lepidopter a: Lymantriidae) nuclear polyhedrosis virus. Environ. Entomol. 24, 1144–1149. doi: 10.1093/ee/24.5.1144
Dhami, M. K., Weir, B. S., Taylor, M. W., and Beggs, J. R. (2013). Diverse honeydew-consuming fungal communities associated with scale insects. PLoS One 8:e70316. doi: 10.1371/journal.pone.0070316
Ellis, M. B., and Ellis, J. P. (1997). Microfungi on Land Plants – An Identification Handbook. London: Croom Helm.
Elsner, G. (2012). Wimmerwuchs an Buche (Fagus sylvatica). Available online at: https://commons.wikimedia.org/wiki/File:Wimmerwuchs.jpg (accessed on 26 May 2020).
Erdtmann, H. (1952). “Phenolic and other extraneous components of coniferous heartwoods; their relation to taxonomy,” in Wood Chemistry, Vol. 1, eds L. E. Wise and E. C. Jahn (New York, NY: Reinhold), 661–688.
Findlay, S. E. G., and Arsuffi, T. L. (1989). Microbial growth and detritus transformations during decomposition of leaf litter in a stream. Freshw. Biol. 21, 261–269. doi: 10.1111/j.1365-2427.1989.tb01364.x
Frangi, J. L., and Lugo, A. E. (1985). Ecosystem dynamics of a subtropical floodplain forest. Ecol. Monogr. 55, 351–369. doi: 10.2307/1942582
Friesen, J. (2020). “Flow pathways of throughfall and stemflow through the subsurface,” in Precipitation Partitioning by Vegetation, eds J. T. Van Stan, E. Gutmann, and J. Friesen (Cham: Springer), 215–228. doi: 10.1007/978-3-030-29702-2_13
Germer, S., Werther, L., and Elsenbeer, H. (2010). Have we underestimated stemflow? Lessons from an open tropical rainforest. J. Hydrol. 395, 169–179. doi: 10.1016/j.jhydrol.2010.10.022
Ghate, S. D., and Sridhar, K. R. (2015a). A new technique to monitor conidia of aquatic hyphomycetes in streams using latex-coated slides. Mycology 6, 161–167. doi: 10.1080/21501203.2015.1110209
Ghate, S. D., and Sridhar, K. R. (2015b). Diversity of aquatic hyphomycetes in sediments of temporary streamlets of Southwest India. Fungal Ecol. 14, 53–61. doi: 10.1016/j.funeco.2014.11.005
Ghate, S. D., and Sridhar, K. R. (2015c). Rain-borne fungi in stemflow and throughfall of six tropical palm species. Czech Mycol. 67, 45–58.
Giacomin, A., and Trucchi, P. (1992). Rainfall interception in a beech coppice (Acquerino, Italy). J. Hydrol. 137, 141–147. doi: 10.1016/0022-1694(92)90052-w
Gmihail. (2014). Acer Platanoides Bark. Available online at: https://commons.wikimedia.org/wiki/File:Acer_platanoides_bark_Bgd.jpg (accessed on 1 October 2020).
Gönczöl, J. (1976). Ecological observations on the aquatic hyphomycetes of Hungary II. Acta Bot. Acad. Scient. Hungar. 22, 51–60.
Gönczöl, J., and Révay, Á (2003). Treehole fungal communities: aquatic, aero-aquatic and dematiaceous hyphomycetes. Fungal Divers. 12, 19–34. doi: 10.1016/j.fbr.2007.02.003
Gönczöl, J., and Révay, Á (2004). Fungal spores in rainwater: stemflow, throughfall and gutter conidial assemblages. Fungal Divers. 16, 67–86.
Gönczöl, J., and Révay, Á (2006). Species diversity of rainborne hyphomycete conidia from living trees. Fungal Divers. 22, 37–54.
Guevara-Escobar, A., González-Sosa, E., Véliz-Chávez, C., Ventura-Ramos, E., and Ramos-Salinas, M. (2007). Rainfall interception and distribution patterns of gross precipitation around an isolated Ficus benjamina tree in an urban area. J. Hydrol. 333, 532–541. doi: 10.1016/j.jhydrol.2006.09.017
Gulis, V., Marvanová, L., and Descals, E. (2007). “An illustrated key to the common temperate species of aquatic hyphomycetes,” in Methods to Study Litter Decomposition: A Practical Guide, eds M. A. S. Graça, F. Bärlocher, and M. O. Gessner (Dordrecht: Springer), 153–168. doi: 10.1007/1-4020-3466-0_21
Gunasekera, S. A., and Webster, J. (1983). Inhibitors of aquatic and aero-aquatic hyphomycetes in pine and oak wood. Trans. Br. Mycol. Soc. 80, 121–125. doi: 10.1016/s0007-1536(83)80172-7
Hanfmampf. (2008). Culm of Phyllostachys vivax f. Aureocaulis. Available online at: https://commons.wikimedia.org/wiki/File:Phyllostachys_vivax_Aureocaulis_culm.JPG (accessed on 26 May 2020).
Havelaar, A. (2020). Stock Photo - Abstract Pattern of Bark on Tiliacordata or Small Leaved Linden. Malaysia: 123RF.
Hughes, S. J. (1958). Revisiones Hyphomycetum aliquot cum appendice de nominibus rejiciendis. Can. J. Bot. 36, 727–836. doi: 10.1139/b58-067
Iida, S., Kakubari, J., and Tanaka, T. (2005). “Litter marks” indicating infiltration area of stemflow-induced water. Tsukuba Geoenviron. Sci 1, 27–31.
Imamura, N., Levia, D. F., Toriyama, J., Kobayashi, M., and Nanko, K. (2017). Stemflow-induced spatial heterogeneity of radiocesium concentrations and stocks in the soil of a broadleaved deciduous forest. Sci. Total Environ. 599, 1013–1021. doi: 10.1016/j.scitotenv.2017.05.017
Ingold, C. T. (1942). Aquatic hyphomycetes of decaying alder leaves. Trans. Br. Mycol. Soc. 25, 339–417. doi: 10.1016/s0007-1536(42)80001-7
Ingold, C. T. (1953). Dispersal in Fungi. Oxford: Claredon Press. doi: 10.1097/00010694-195311000-00014
Ingold, C. T. (1966). The tetraradiate aquatic fungal spore. Mycologia 58, 43–56. doi: 10.2307/3756987
Ingold, C. T. (1975). An illustrated guide to aquatic and water-borne hyphomycetes (Fungi Imperfecti) with notes on their biology. Freshw. Biol. Assoc. Sci. Publ. 30, 1–96.
Jakus, R., and Blazenec, M. (2011). Treatment of bark beetle attacked trees with entomopathogenic fungus Beauveria bassiana (Balsamo) Vuillemin. Folia For. Polon. Ser. A For. 53, 150–155.
Johnson, M. S., and Jost, G. (2011). “Ecohydrology and biogeochemistry of the rhizosphere in forested ecosystems,” in Forest hydrology and Biogeochemistry, eds D. Levia, D. Carlyle-Moses, and T. Tanaka (Dordrecht: Springer), 483–498. doi: 10.1007/978-94-007-1363-5_24
Karamchand, K. S., and Sridhar, K. R. (2008). Water-borne conidial fungi inhabiting tree holes of the west coast and Western Ghats of India. Czech Mycol. 60, 63–74. doi: 10.33585/cmy.60105
Kendrick, B. (1990). “Fungal allergens,” in Sampling and Identifying Allergenic Pollens and Moulds, ed. E. G. Smith (San Antonio, TX: Blewstone Press), 41–165.
Klučiarová, D., Márton, P., Pichler, V., Márton, E., and Túnyi, I. (2008). Pollution detection by magnetic susceptibility measurements aided by the stemflow effect. Water Air Soil Pollut. 189, 213–223. doi: 10.1007/s11270-007-9569-8
Koch, K., Bhushan, B., and Barthlott, W. (2008). Diversity of structure, morphology and wetting of plant surfaces. Soft Matter 4, 1943–1963. doi: 10.1039/b804854a
Krämer, I., and Hölscher, D. (2009). Rainfall partitioning along a tree diversity gradient in a deciduous old-growth forest in Central Germany. Ecohydrology 2, 102–114. doi: 10.1002/eco.44
Kwiecień, A. (2005). Piceaabies Bark, Poland, Near Wrocław. Available online at: https://commons.wikimedia.org/wiki/File:Picea_abies_bark.jpg (accessed on 26 May 2020).
Lambais, M. R., Lucheta, A. R., and Crowley, D. E. (2014). Bacterial community assemblages associated with the phyllosphere, dermosphere, and rhizosphere of tree species of the Atlantic forest are host taxon dependent. Microb. Ecol. 68, 567–574. doi: 10.1007/s00248-014-0433-2
Lefnaer, S. (2016). Quercus cerris SL2. Available online at: https://commons.wikimedia.org/wiki/File:Quercus_cerris_sl2.jpg (accessed on 26 May 2020).
Levia, D. F., and Germer, S. (2015). A review of stemflow generation dynamics and stemflow-environment interactions in forests and shrublands. Rev. Geophys. 53, 673–714. doi: 10.1002/2015rg000479
Levia, D. F., Van Stan, J. T., Siegert, C. M., Inamdar, S. P., Mitchell, M. J., Mage, S. M., et al. (2011). Atmospheric deposition and corresponding variability of stemflow chemistry across temporal scales in a mid-Atlantic broadleaved deciduous forest. Atmos. Environ. 45, 3046–3054. doi: 10.1016/j.atmosenv.2011.03.022
Louveaux, J., Maurizio, A., and Vorwohl, G. (1978). Methods of melissopalynology. Bee World 59, 139–157. doi: 10.1080/0005772x.1978.11097714
MacKinnon, J. A. (1982). Stemflow and Throughfall Mycobiota of a Trembling Aspen-Red Alder Forest. Doctoral dissertation. Vancouver, BC: University of British Columbia.
Magliano, P. N., Whitworth-Hulse, J. I., and Baldi, G. (2019). Interception, throughfall and stemflow partition in drylands: global synthesis and meta-analysis. J. Hydrol. 568, 638–645. doi: 10.1016/j.jhydrol.2018.10.042
Magyar, D. (2007). “Aeromycological aspects of mycotechnology,” in Mycotechnology: Current Trends and Future Prospects, ed. M. K. Rai (New Delhi: I.K. International Publishing House), 226–263.
Magyar, D., and Révay, Á (2008). Trinacriumtothii spec. nov. (Hyphomycetes) from the cortex of living tree. Nova Hedwigia 87, 513–519. doi: 10.1127/0029-5035/2008/0087-0513
Magyar, D., and Révay, Á (2009a). New species of Oncopodiella (Hyphomycetes) from living trees. Nova Hedwigia 88, 169–182. doi: 10.1127/0029-5035/2009/0088-0169
Magyar, D., and Révay, Á (2009b). Oncopodium elaeagni, a new hyphomycete from Hungary. Nova Hedwigia 88, 475–481. doi: 10.1127/0029-5035/2009/0088-0475
Magyar, D., Gönczöl, J., Révay, Á, Grillenzoni, F., and Seijo-Coello, M. D. C. (2005). Stauro- and scolecoconidia in floral and honeydew honeys. Fungal Divers. 20, 103–120.
Magyar, D., Merényi, Z., Bratek, Z., Baral, H.-O., and Marson, G. (2016a). Lecophagus vermicola sp. nov., a nematophagous hyphomycete with an unusual hunting strategy. Mycol. Progress 15, 1137–1144. doi: 10.1007/s11557-016-1235-3
Magyar, D., Merényi, Z., Körmöczi, P., Bratek, Z., and Kredics, L. (2017a). Phylogenetic analysis and description of two new species of pollen-parasitic Retiarius (anamorphic Orbiliomycetes). Nova Hedwigia 105, 411–423. doi: 10.1127/nova_hedwigia/2017/0420
Magyar, D., Merényi, Z., Udvardy, O., Kajtor-Apatini, D., Körmöczi, P., Fülöp, A., et al. (2018). Mycoceros antennatissimus gen. et sp. nov.: a mitosporic fungus capturing pollen grains. Mycol. Progress 17, 33–43. doi: 10.1007/s11557-017-1275-3
Magyar, D., Mura-Mészáros, A., and Grillenzoni, F. (2016b). Fungal diversity in floral and honeydew honeys. Acta Bot. Hungar. 58, 145–166. doi: 10.1556/034.58.2016.1-2.6
Magyar, D., Vass, M., and Oros, G. (2017b). Dendrotelmata (water-filled tree holes) as fungal hotspots-a long term study. Crypt. Mycol. 38, 55–66. doi: 10.7872/crym/v38.iss1.2017.55
Mali, S. S., Sarkar, P. K., Naik, S. K., Singh, A. K., and Bhatt, B. P. (2020). Predictive models for stemflow and throughfall estimation in four fruit tree species under hot and sub-humid climatic region. Hydrol. Res. 51, 47–64. doi: 10.2166/nh.2019.052
Marvanová, L. (1997). “Freshwater hyphomycetes: a survey with remarks on tropical taxa,” in Tropical Mycology, eds K. K. Janardhanan, C. Rajendran, K. Natarajan, and D. L. Hawksworth (New York, NY: Science Publishers), 169–226.
McPherson, J. R. (2018). Eucalyptus grandis, The Flooded Gum or Rose Gum, Has a Few Ornamentally Planted Specimens in 7th Brigade Park. Available online at: https://commons.wikimedia.org/wiki/File:Eucalyptus_grandis_stem_flow_lines_7th_Brigade_Park_Chermside_L1100312.jpg (accessed on 1 October 2020).
Mella, V. S., Orr, C., Hall, L., Velasco, S., and Madani, G. (2020). An insight into natural koala drinking behaviour. Ethology 126, 858–863. doi: 10.1111/eth.13032
Mendieta-Leiva, G., Porada, P., and Bader, M. Y. (2020). “Interactions of epiphytes with precipitation partitioning,” in Precipitation Partitioning by Vegetation, eds J. Van Stan II, E. Gutmann and J. Friesen (Cham: Springer), 133–146. doi: 10.1007/978-3-030-29702-2_9
Miller, W. B., Peralta, E., Ellis, D. R., and Perkins, H. H. Jr. (1994). Stickiness potential of individual insect honeydew carbohydrates on cotton lint. Text. Res. J. 64, 344–350. doi: 10.1177/004051759406400606
Mitscherlich, G. (1981). Wald, Wachstum und Umwelt. 2. Band: Waldklima und Wasserhaushalt. Frankfurt am Main: Sauerländer’s Verlag.
Mosello, R., Brizzio, C. R., Kotzias, D., Marchetto, A., Rembges, D., and Tartari, G. (2002). The chemistry of atmospheric deposition in Italy in the framework of the National Programme for Forest Ecosystems Control (CONECOFOR). J. Limnol. 61, 77–92. doi: 10.4081/jlimnol.2002.s1.77
Ndakara, O. E. (2012). Biogeochemical consequences of hydrologic conditions in isolated stands of terminalia cattapa in the rainforest zone of Southern Nigeria. Spec. Publ. Niger. Assoc. Hydrol. Sci. 1, 134–144. doi: 10.4314/ejesm.v5i1.1
Ndakara, O. E. (2016). Hydrological nutrient flux in isolated exotic stands of Mangifera Indica Linn: implications for sustainable rainforest ecosystem management in South-Southern Nigeria. Niger. J. Sci. Environ. 14, 125–131.
Nizinski, J. J., Galat, G., and Galat-Luong, A. (2011). Water balance and sustainability of eucalyptus plantations in the Kouilou basin (Congo-Brazzaville). Russ. J. Ecol. 42, 305–314. doi: 10.1134/s1067413611040126
Nowak, D. J., Hirabayashi, S., Bodine, A., and Hoehn, R. (2013). Modeled PM2. 5 removal by trees in ten US cities and associated health effects. Environ. Pollut. 178, 395–402. doi: 10.1016/j.envpol.2013.03.050
Peck, A. K. (2004). Hydrometeorologische und Mikroklimatische Kennzeichen von Buchenwäldern. PhD thesis. Freiburg im Breisgau: University of Freiburg.
Ponette-González, A. G., Van Stan, J. T., and Magyar, D. (2020). “Things seen and unseen in throughfall and stemflow,” in Precipitation Partitioning by Vegetation, eds J. Van Stan II, E. Gutmann and J. Friesen (Cham: Springer), 71–88. doi: 10.1007/978-3-030-29702-2_5
Proctor, H. C., Montgomery, K. M., Rosen, K. E., and Kitching, R. L. (2002). Are tree trunks habitats or highways? A comparison of oribatid mite assemblages from hoop-pine bark and litter. Austr. J. Entomol. 41, 294–299. doi: 10.1046/j.1440-6055.2002.00309.x
Ptatscheck, C., Milne, P. C., and Traunspurger, W. (2018). Is stemflow a vector for the transport of small metazoans from tree surfaces down to soil? BMC Ecol. 18:43. doi: 10.1186/s12898-018-0198-4
Pypker, T. G., Levia, D. F., Staelens, J., and Van Stan, J. T. (2011). “Canopy structure in relation to hydrological and biogeochemical fluxes,” in Forest Hydrology and Biogeochemistry, eds D. Levia, D. Carlyle-Moses, and T. Tanaka (Dordrecht: Springer), 371–388. doi: 10.1007/978-94-007-1363-5_18
Ranzoni, F. V. (1956). The perfect state of Flagellosporapenicillioides. Am. J. Bot. 43, 13–17. doi: 10.1002/j.1537-2197.1956.tb10456.x
Rashid, N. S. A., and Askari, M. (2014). ““Litter marks” around oil palm tree base indicating infiltration area of stemflow-induced water,” in Proceedings of the National Seminar on Civil Engineering Research, Johor Bahru.
Ray, M. P. (1970). Preliminary observations on stem-flow, etc., in Alstonia scholaris and Shorea robusta plantations at Arabari, West Bengal. Indian For. 96, 482–493.
Révay, Á, and Gönczöl, J. (2010). Rainborne hyphomycete conidia from evergreen trees. Nova Hedwigia 91, 151–163. doi: 10.1127/0029-5035/2010/0091-0151
Révay, Á, and Gönczöl, J. (2011a). Aquatic hyphomycetes and other water-borne fungi in Hungary. Czech Mycol. 63, 133–151. doi: 10.33585/cmy.63203
Révay, Á, and Gönczöl, J. (2011b). Canopy fungi (“terrestrial aquatic hyphomycetes”) from twigs of living evergreen and deciduous trees in Hungary. Nova Hedwigia 92, 303–316. doi: 10.1127/0029-5035/2011/0092-0303
Rosier, C. L., Moore, L. D., Wu, T., and Van Stan, J. T. (2015). Forest canopy precipitation partitioning: an important plant trait Influencing the spatial structure of the symbiotic soil microbial community. Adv. Bot. Res. 75, 215–240. doi: 10.1016/bs.abr.2015.09.005
Sadeghi, S. M. M., Gordon, D. A., and Van Stan, J. T. II (2020). “A global synthesis of throughfall and stemflow hydrometeorology,” in Precipitation Partitioning by Vegetation, eds J. Van Stan II, E. Gutmann and J. Friesen (Cham: Springer), 49–70. doi: 10.1007/978-3-030-29702-2_4
Sahu, M. L., Seth, N. K., and Upadhyaya, D. (2006). Exploratory studies on rainfall partitioning in block plantations of social forestry trees in central India. J. Trop. For. 22, 12–15.
Salemi, L. F. (2019). Turning rain into internal precipitation and stemflow: observations of palm trees in a urban environment. Cerrado Agroc. Rev. Centre Univ. Pato de Minas 10, 45–50. doi: 10.33585/cmy.67106
Salicyna. (2017). Metasequoia Glyptostroboides, Marszewo Forest. Available online at: https://commons.wikimedia.org/wiki/File:Metasequoia_glyptostroboides_2017-05-16_0376.jpg (accessed on 26 May 2020).
Sansalone, J. J., and Cristina, C. M. (2004). First flush concepts for suspended and dissolved solids in small impervious watersheds. J. Environ. Eng. 130, 1301–1314. doi: 10.1061/(asce)0733-9372(2004)130:11(1301)
Scheffer, T. C., and Cowling, E. B. (1966). Natural resistance of wood to microbial deterioration. Ann. Rev. Phytopathol. 4, 147–170. doi: 10.1146/annurev.py.04.090166.001051
Schooling, J. T., and Carlyle-Moses, D. E. (2015). The influence of rainfall depth class and deciduous tree traits on stemflow production in an urban park. Urban Ecosyst. 18, 1261–1284. doi: 10.1007/s11252-015-0441-0
Selosse, M., Vohník, M., and Chauvet, E. (2008). Out of the rivers: are some aquatic hyphomycetes plant endophytes? New Phytol. 178, 3–7. doi: 10.1111/j.1469-8137.2008.02390.x
Serrano, R. C. (1982). Hydrology of Different Coconut (Cocos nucifera L.)-based agroecosystems. Los Baños: University of the Philippines.
Shaaban, B., Seeburger, V., Schroeder, A., and Lohaus, G. (2020). Sugar, amino acid and inorganic ion profiling of the honeydew from different hemipteran species feeding on Abies alba and Piceaabies. PLoS One 15:e0228171. doi: 10.1371/journal.pone.0228171
Sivichai, S., and Jones, E. B. G. (2003). “Teleomorphic-anamorphic connections of freshwater fungi,” in Freshwater Mycology. Fungal Diversity Series, eds C. K. M. Tsui and K. D. Hyde (Ottawa: National Museum of Sciences), 259–274.
Sokolski, S., Piché, Y., Chauvet, E., and Bérubé, J. A. (2006). A fungal endophyte of black spruce (Picea mariana) needles is also an aquatic hyphomycete. Mol. Ecol. 15, 1955–1962. doi: 10.1111/j.1365-294x.2006.02909.x
Sridhar, K. R. (2009). Fungi in the Tree Canopy–An Appraisal. Applied Mycology. Wallingford: CAB International, 73–91. doi: 10.1079/9781845935344.0073
Sridhar, K. R., and Bärlocher, F. (1993). Aquatic hyphomycetes on leaf litter in and near a stream in Nova Scotia, Canada. Mycol. Res. 97, 1530–1535. doi: 10.1016/s0953-7562(09)80229-3
Sridhar, K. R., and Karamchand, K. S. (2009). Diversity of water-borne fungi in stemflow and throughfall of tree canopies in India. Sydowia 61, 327–344.
Sridhar, K. R., Karamchand, K. S., and Bhat, R. (2006). Arboreal water-borne hyphomycetes on oak-leaf basket fern Drynaria quercifolia. Sydowia-Horn- 58, 309–320.
Sridhar, K. R., Nagesh, H., and Sharathchandra, K. (2020). Assemblage and diversity of asexual fungi in 10 terrestrial damp leaf litters: comparison of two incubation techniques. Asian J. Mycol. 3, 362–375. doi: 10.5943/ajom/3/1/10
Starr, K., and Starr, F. (2009). Coccothrinaxbarbadensis Bark at Kahului, Maui, Hawaii. Available online at: https://commons.wikimedia.org/wiki/File:Starr-090806-4046-Coccothrinax_barbadensis-bark-Kahului-Maui_(24344960903).jpg (accessed on 26 May 26 2020).
Steubing, L., and Kirschbaum, U. (1976). Immissionsbelastung der Strassenrandvegetation. Nat. Landschaft. 51, 239–244.
Stubbins, A., Guillemette, F., and Van Stan, J. T. (2020). “Throughfall and stemflow: the crowning headwaters of the aquatic carbon cycle,” in Precipitation Partitioning by Vegetation, eds J. Van Stan II, E. Gutmann and J. Friesen (Cham: Springer), 121–132. doi: 10.1007/978-3-030-29702-2_8
Sudheep, N. M., and Sridhar, K. R. (2010). Water-borne hyphomycetes in tree canopies of Kaiga (Western Ghats), India. Acta Mycol. 45, 185–195. doi: 10.5586/am.2010.024
Suzuki, K. (2006). Characterisation of airborne particulates and associated trace metals deposited on tree bark by ICP-OES, ICP-MS, SEM-EDX and laser ablation ICP-MS. Atmos. Environ. 40, 2626–2634. doi: 10.1016/j.atmosenv.2005.12.022
Tanaka, N., Levia, D., Igarashi, Y., Yoshifuji, N., Tanaka, K., Tantasirin, C., et al. (2017). What factors are most influential in governing stemflow production from plantation-grown teak trees? J. Hydrol. 544, 10–20. doi: 10.1016/j.jhydrol.2016.11.010
Tanaka, T., Tsujimura, M., and Taniguchi, M. (1991). “Infiltration area of stemflow-induced water,” in Annual Report-Institute of Geoscience (Tsukuba: University of Tsukuba), 30–32.
Tischer, A., Michalzik, B., and Lotze, R. (2020). Nonuniform but highly preferential stemflow routing along bark surfaces and actual smaller infiltration areas than previously assumed: a case study on European beech (Fagus sylvatica L.) and sycamore maple (Acer pseudoplatanus L.). Ecohydrology 13:e2230. doi: 10.1002/eco.2230
Tubaki, K., Tokumasu, S., and Anclo, K. (1985). Morning dew and Tripospermum (Hyphomycetes). Bot. J. Linnean Soc. 91, 45–50. doi: 10.1111/j.1095-8339.1985.tb01133.x
Tucker, A., Levia, D. F., Katul, G. G., Nanko, K., and Rossi, L. F. (2020). A network model for stemflow solute transport. Appl. Math. Model. 88, 266–282. doi: 10.1016/j.apm.2020.06.047
Uroz, S., Buee, M., Deveau, A., Mieszkin, S., and Martin, F. (2016). Ecology of the forest microbiome: highlights of temperate and boreal ecosystems. Soil Biol. Biochem. 103, 471–488. doi: 10.1016/j.soilbio.2016.09.006
Valová, M., and Bieleszová, S. (2008). Interspecific variations of bark’s water storage capacity of chosen types of trees and the dependence on occurrence of epiphytic mosses. GeoSci. Eng. 54, 45–51.
Van Stan, J. T., and Allen, S. T. (2020). What we know about stemflow’s infiltration area. Front. For. Glob. Change 3:61. doi: 10.3389/ffgc.2020.00061
Van Stan, J. T., and Gordon, D. A. (2018). Mini-review: stemflow as a resource limitation to near-stem soils. Front. Plant Sci. 9:248. doi: 10.3389/fpls.2018.00248
Van Stan, J. T., and Levia, D. F. (2010). Inter-and intraspecific variation of stemflow production from Fagus grandifoliaEhrh.(American beech) and Liriodendron tulipifera L.(yellow poplar) in relation to bark microrelief in the eastern United States. Ecohydrology 3, 11–19. doi: 10.1002/eco.83
Van Stan, J. T., Hildebrandt, A., Friesen, J., Metzger, J. C., and Yankine, S. A. (2020a). “Spatial variability and temporal stability of local net precipitation patterns,” in Precipitation Partitioning by Vegetation, eds J. Van Stan II, E. Gutmann and J. Friesen (Cham: Springer), 89–104. doi: 10.1007/978-3-030-29702-2_6
Van Stan, J. T., Lewis, E. S., Hildebrandt, A., Rebmann, C., and Friesen, J. (2016). Impact of interacting bark structure and rainfall conditions on stemflow variability in a temperate beech-oak forest, central Germany. Hydrol. Sci. J. 61, 2071–2083. doi: 10.1080/02626667.2015.1083104
Van Stan, J. T., Morris, C. E., Aung, K., Kuzyakov, Y., Magyar, D., Rebollar, E. A., et al. (2020b). “Precipitation partitioning—hydrologic highways between microbial communities of the plant microbiome?,” in Precipitation Partitioning by Vegetation, eds J. Van Stan II, E. Gutmann and J. Friesen (Cham: Springer), 229–252. doi: 10.1007/978-3-030-29702-2_14
Van Stan, J. T., Ponette-Gonzalez, A. G., Swanson, T., and Weathers, K. C. (2021). Concepts and questions: throughfall and stemflow are major hydrologic highways for particulate traffic through tree canopies. Front. Ecol. Environ. (in press).
Van Stan, J. T., Wagner, S., Guillemette, F., Whitetree, A., Lewis, J., Silva, L., et al. (2017). Temporal dynamics in the concentration, flux, and optical properties of tree-derived dissolved organic matter in an epiphyte-laden oak-cedar forest. J. Geophys. Res. Biogeosci. 122, 2982–2997. doi: 10.1002/2017jg004111
Vass, M. (2015). Hydrobiological Properties and Ecology of Fungal Consortia of Dendrotelmata. Ms.C. thesis. Veszprém: Pannon University.
Vass, M., Révay, Á, Kucserka, T., Hubai, K., Üveges, V., Kovács, K., et al. (2013). Aquatic hyphomycetes as survivors and/or first colonizers after a red sludge disaster in the Torna stream, Hungary. Int. Rev. Hydrobiol. 98, 217–224. doi: 10.1002/iroh.201301540
Vulinoviæ, J. N., Loliæ, S. B., Vujèiæ, S. B., and Matavulj, M. N. (2019). Schizophyllum commune–the dominant cause of trees decay in alleys and parks in the City of Novi Sad (Serbia). Biol. Serb. 40, 26–33.
Webster, J., and Descals, E. (1979). “The teleomorphs of water-borne hyphomycetes from freshwater,” in The Whole Fungus, ed. B. Kendrick (Ottawa: National Museums of Canada), 419–447.
West, J. (2010). Nature Notes: Tree Foam. Available online at: http://ramblinghemlock.blogspot.com/2010/09/first-website.html (accessed 1 October 2020) Google Scholar
Xu, X., Yu, X., Mo, L., Xu, Y., Bao, L., and Lun, X. (2019). Atmospheric particulate matter accumulation on trees: a comparison of boles, branches and leaves. J. Clean. Prod. 226, 349–356. doi: 10.1016/j.jclepro.2019.04.072
Keywords: fungi, conidia, spores, honeydew, bark, cortisphere, phyllosphere
Citation: Magyar D, Van Stan JT II and Sridhar KR (2021) Hypothesis and Theory: Fungal Spores in Stemflow and Potential Bark Sources. Front. For. Glob. Change 4:623758. doi: 10.3389/ffgc.2021.623758
Received: 30 October 2020; Accepted: 04 March 2021;
Published: 25 March 2021.
Edited by:
Daniel Limehouse McLaughlin, Virginia Tech, United StatesReviewed by:
Mark B. Green, Case Western Reserve University, United StatesKasem Soytong, King Mongkut’s Institute of Technology Ladkrabang, Thailand
Copyright © 2021 Magyar, Van Stan and Sridhar. This is an open-access article distributed under the terms of the Creative Commons Attribution License (CC BY). The use, distribution or reproduction in other forums is permitted, provided the original author(s) and the copyright owner(s) are credited and that the original publication in this journal is cited, in accordance with accepted academic practice. No use, distribution or reproduction is permitted which does not comply with these terms.
*Correspondence: Donát Magyar, magyar.donat@gmail.com